Hypothesis 1 (granites typically contain solid restite)
Some petrologists have contended that granitic magmas are rarely entirely liquid, but instead are mixtures of minimum or near-minimum temperature (‘haplogranitic’) liquid, together with the unmelted remainder of the source-rock (solid ‘restite’), which is transported from the source to the emplacement level [e.g., Chappell and White, 1974, 1976, 1991; Chappell, 1984, 2004; White and Chappell, 1977, 1988; Griffin et al., 1978; Hine et al., 1978; Chappell et al., 1987, 1993, 2000; Wyborn et al., 1981; Chappell and Wyborn, 2004]. According to this hypothesis, the solid restite contains most of the calcic and mafic components of the resulting granitoids and equivalent felsic volcanic rocks. The ‘solid restite hypothesis’ encounters the following seven main problems.
(1) Problem of biotite and hornblende: In its simplest form, the solid restite hypothesis regards biotite and hornblende crystals in granitoids as being transported from the source, which implies (a) relatively low-temperature melting, in order to preserve the biotite and hornblende, and (b) externally derived water to produce the liquid, because internal water is retained in the biotite and hornblende. However, field and petrological evidence in many migmatite complexes, as well as experimental evidence, indicate that incongruent melting of biotite and hornblende is the main process responsible for the formation of crustal granitic magmas, producing hydrous liquid and anhydrous or weakly hydrous (‘peritectic’) solids, such as pyroxene, garnet or cordierite. This destruction of source biotite and hornblende necessitates that all or most of the biotite and hornblende in granitoids is magmatic, unless it can be shown that they form by replacement of anhydrous restite crystals without the restite chemical components dissolving in the liquid and re-precipitating as new crystals, which would be magmatic.
(2) Lack of convincing microstrructural evidence in inferred restite crystals and aggregates: Sillimanite inclusions in cordierite in some S-type granites have been cited as indicators of restite [e.g., White and Chappell, 1977], but they can also be explained by an accidental xenocrystic origin for the cordierite [e.g., Vernon, 2007]. Moreover, inclusions of magmatic sillimanite occur in cordierite and muscovite phenocrysts in some peraluminous dacites [Zeck, 1972; Roycroft, 1991; Büttner, 2005]. The solid restite hypothesis contends that the upper amphibolite facies metasedimentary enclaves in many S-type granitoids are restite, but they could be accidental xenoliths from an amphibolite facies terrane above the granulite facies source terrane [e.g., Vernon, 2007].
Corroded, unzoned cores conceivably could represent transported solids that were partly dissolved in the magma in the source rocks or during ascent. Examples of crystals with cores and mantles in granitoids are (i) mantled quartz and feldspar xenocrysts (‘antecrysts’) resulting from magma mixing, (ii) rapakivi structure, which commonly results from magma mixing or pressure change, (iii) orthopyroxene mantled by biotite, clinopyroxene partly replaced by hornblende, and garnet partly replaced by cordierite ± biotite, the cores of which could all represent magmatic precipitates, restite or xenocrysts (see below); and (iv) calcic plagioclase cores in some granitoids, which have been inferred to be transported restite [e.g., Chappell et al., 1987], but which also can be explained as products of fractional crystallization with variation of a(H2O) [Allen, 2001]. None of these types of core necessitates explanation by transport from the source, which emphasizes the general lack of convincing microstructural evidence for restite crystals in granitoids.
As noted above, rims of biotite on orthopyroxene or hornblende on clinopyroxene (Figure 8) may or may not represent partly replaced restite, it being difficult to distinguish between phenocrystic, xenocrystic and restitic origins of the pyroxene. However, even if replaced restite is inferred, it is generally difficult to determine whether the original grain was replaced in the solid state, retaining its former shape, or whether it was dissolved in the melt and the new mineral magmatically precipitated on the dissolving grain. Stevens et al. [2007] inferred that peritectic garnet grains were carried up rapidly from source metapelites in peraluminous granitic magmas responsible for the Cape Granite Suite, South Africa. As a result of abrupt decrease in pressure, the garnet broke down to lower-pressure minerals, such as cordierite ± orthopyroxene, releasing the components of these minerals into the liquid, from which they precipitated as magmatic minerals. Villaros et al. [2009] suggested that peritectic garnet from the source underwent coupled dissolution and re-precipitation during ascent and cooling of leucogranite magma. Villaros et al. [2009] inferred that the garnet crystals retained their original shapes through this process, which implies that the silicate liquid thoroughly penetrated the crystals, dissolving them and simultaneously precipitating new garnet, as proposed by Putnis [2002, p. 695] for reactions between plagioclase crystals and silicate liquid. However, it is difficult to eliminate the possibility of dissolution and independent crystallization of new magmatic crystals, in view of the lack of primary inclusions and the common subhedral shapes of the garnet crystals in these rocks. Similarly, despite the presentation of abundant detailed evidence of the entrainment of peritectic garnet in leucogranitic magma by Taylor and Stevens [2010], the garnet grains show evidence of (1) precipitation of compositionally identical garnet as inclusion-free rims on inferred restite cores, and (2) grain “amalgamation” or “fusion”, which presumably involves some dissolution and re-precipitation, and (3) increasing magmatic character (e.g., euhedral shape) of the garnet with increasing degree of segregation of magma from the source. These processes tend to blur the structural distinction between incorporated solid restite (even if compositionally modified) and magmatic precipitation of dissolved restite components.
Cordierite with oscillatory zoning in some granitoids [Erdmann et al., 2009] and peraluminous felsic volcanic rocks [Zeck, 1972] is consistent with magmatic precipitation of dissolved components of former restite, resister (unreactive material in the source rock) or xenocrystic cordierite and/or other aluminous minerals.
(3) Microgranitoid enclaves are igneous: Microgranitoid enclaves (‘mafic enclaves’) in granitoids have been interpreted as restite or modified restite. [e.g., Chappell, 1996, p. 467]. However, the porphyritic and fine-grained igneous microstructures of microgranitoid enclaves (Figures 9, 10, 11, 12) are inconsistent with a restite origin. Characteristic igneous features of microgranitoid enclaves [e.g., Vernon, 1983, 1984, 1990, 1991, 2004, 2007] include euhedral plagioclase phenocrysts with oscillatory zoning, abundant zoned plagioclase laths, magmatic flow foliations marked by aligned plagioclase laths, interstitial quartz or K-feldspar in a poikilitic relationship with plagioclase and/or biotite, highly elongate orthopyroxene crystals, thin platy biotite crystals, acicular apatite and ilmenite, and skeletal zircon, as well as features characteristic of magma mixing, such as mantled quartz and feldspar xenocrysts [e.g., Vernon, 2007], as shown in Figures 11 and 13. The microstructures of the enclaves are similar to those of many dykes (Figures 14, 15), which are unquestionably igneous, as noted by Harker and Marr [1891]. Moreover, identical enclaves occur in metaluminous and peraluminous volcanic rocks (Figure 16), some of these enclaves being glassy, which confirms that they were originally magma globules, not restite [e.g., Vernon, 1983, 1984, 1991, 2004]. In addition, miaroles filled with euhedral feldspar crystals projecting inwards into quartz [Vernon, 1991, figure 11], as shown in Figure 17, are clear evidence of the escape of gas from magma prior to solidification of the enclave [e.g., Vernon, 1983, p. 98; 1991, pp. 289-290].
Figure 9. Igneous microstructure of microgranitoid enclave
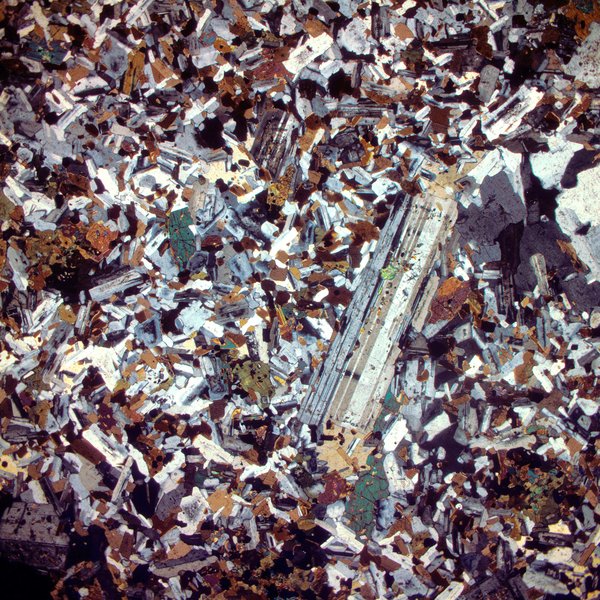
Microtonalite enclave in the Sierra Nevada Batholith, California, USA, consisting of phenocrysts of zoned plagioclase in a groundmass of elongate plagioclase, hornblende and biotite with interstitial quartz. Crossed polars; base of photo 7 mm.
Figure 10. Plagioclase and hornblende phenocrysts in microgranitoid enclave
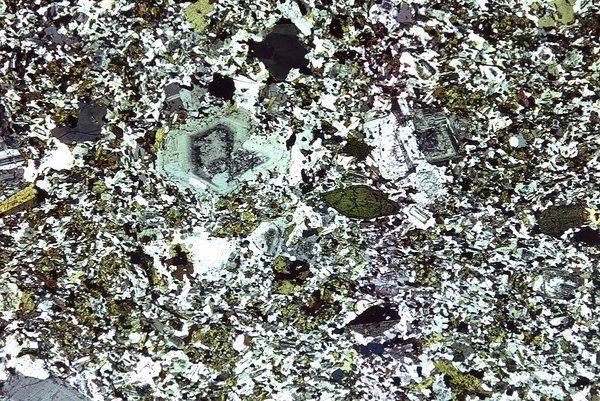
Microgranodiorite enclave, Moonbi Supersuite, New England Batholith, New South Wales, Australia, consisting of euhedral phenocrysts of hornblende and zoned plagioclase, in a fine-grained groundmass of plagioclase laths, biotite, hornblende and quartz. The plagioclase laths locally show a magmatic flow alignment. Crossed polars; base of photo 8.4 mm.
Figure 11. Poikilitic structure in microgranitoid enclave
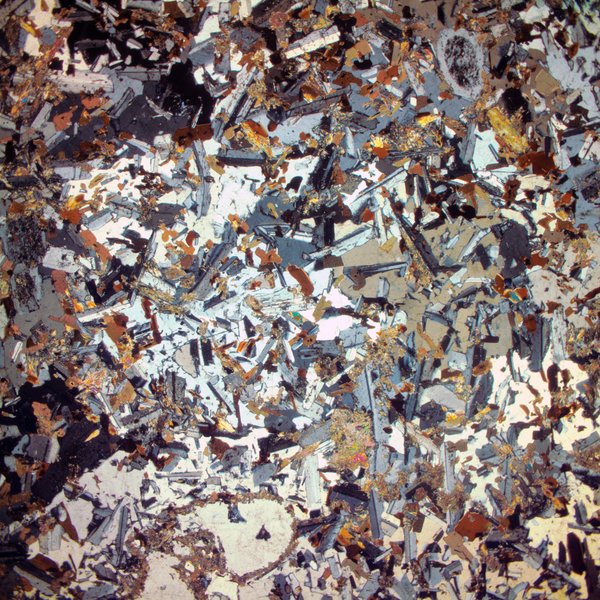
Peraluminous microtonalite enclave, Cowra Granodiorite New South Wales, Australia, with an igneous microstructure, consisting mainly of plagioclase, biotite and orthopyroxene (mostly altered to hydrothemral fine-grained amphibole aggregates), with interstitial quartz, which has a poikilitic relationship with the other minerals. Also shown (bottom) is a quartz xenocryst (“ocellus”) with a rim of fine-grained orthopyroxene (later altered), reflecting magma mixing. Crossed polars; base of photo 7 mm.
The magmatic nature of microgranitoid enclaves is also confirmed by K-feldspar megacrystic xenocrysts with overgrowths containing random, small inclusions of groundmass minerals, adjacent to aligned, larger crystals in the enclave groundmass (Vernon, 1990, fig. 6a), showing that minerals in the groundmass continued to grow, and the enclave magma underwent magmatic flow, after crystallization of the overgrowths.
In an attempt to account for the igneous microstructures, Chen et al. [1989], Chappell and White [1991], White et al. [1991] and White et al. [1999] speculated that these putative “restite” enclaves contained a small amount of melt, which somehow converted a metamorphic into an igneous-looking microstructure, which White et al. [1991] called “pseudo-doleritic.” The mechanisms of this questionable transformation were not explained. Crystallization of magma is a simpler and more probable interpretation, even for peraluminous orthopyroxene-biotite microtonalite enclaves in peraluminous granitoids, such as the Cowra Granodiorite, New South Wales, Australia, which show all the igneous features listed above [e.g., Vernon, 2007].
Figure 12. Magmatic flow structure in microgranitoid enclave
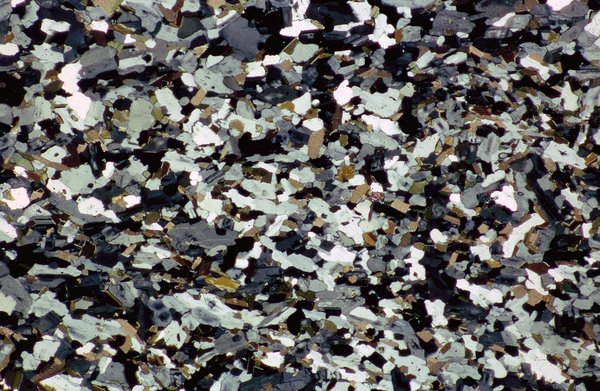
Microgranodiorite enclave, Tioga Pass, Sierra Nevada Batholith, California, USA, consisting mainly of plagioclase, quartz, biotite and hornblende. Many of the biotite grains show {001} crystal faces, and some plagioclase grains also show crystal faces. Most plagioclase/plagioclase and plagioclase/quartz interfaces are indented, owing probably to mutual impingement, though possibly in response to minor deformation. The elongate biotite and plagioclase grains show a moderate to strong alignment, reflecting magmatic flow. Crossed polars; base of photo 3.3 mm.
Waight et al. [2001] and Nicholls et al. [2001] showed that peraluminous microgranitoid enclaves in Lachlan Fold Belt (SE Australia) peraluminous granitoids have isotopic compositions (initial 87Sr/86Sr and εNd) that are generally more primitive than, or similar to, those of the host granitoid, and that the high CaO contents and relatively high εNd of the enclaves are not found in either the Palaeozoic Lachlan Fold Belt turbidites or the metapsammitic-pelitic enclaves in Lachlan Fold Belt granitoids. The isotopic variability, lower initial 87Sr/86Sr and higher εNd of the enclaves, compared with the host granitoid, are probably the result of equilibration of the enclave magma with an initially isotopically more primitive magma [Waight et al., 2001], presumably during magma mixing at depth, before the resulting hybrid magma entered the granitoid pluton and disaggregated into enclaves.
(4) Crystal-poor felsic feeder dykes: Good evidence of the absence of restite in granitoid magmas is provided by crystal-free or crystal-poor felsic feeder dykes that enter and become dispersed into a granitoid pluton, as in the Kameruka pluton of the Bega Batholith, south-eastern New South Wales, Australia [Collins et al., 2006].
(5) Separation of crystals and liquid: When a small amount of silicate liquid (“melt”) is formed, it tends to escape from the source rock [e.g., Bons, 2007; Bons et al., 2004, 2008], leaving most of the solid material behind. Moreover, solids tend to separate from liquids during flow [e.g., Petford and Koenders, 1998]. Therefore, if both crystals and liquid move as a mass (forming a “diatexite”), crystals tend to separate and form layer concentrations or “schlieren” [Sawyer, 1998; Milord and Sawyer, 2003] that tend to coalesce and be left behind [e.g., Vernon, 2007, p. 171; Vernon and Clarke, 2008, pp. 182-184]. This separation commonly occurs close to the source [e.g., Sawyer, 1996, 1998; Sawyer et al., 1999].
Figure 13. Evidence of magma mixing in enclave
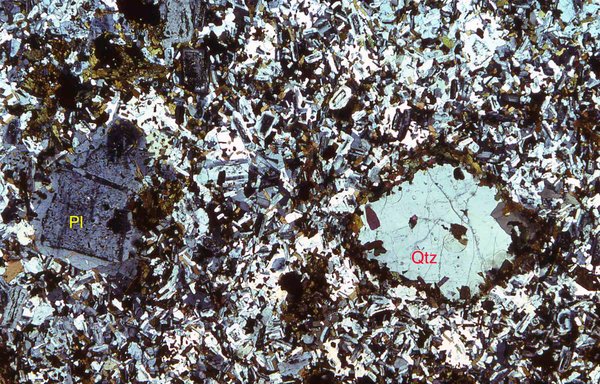
Microgranodiorite enclave, Moonbi Supersuite, New England Batholith, New South Wales, Australia, containing a phenocryst of plagioclase (Pl) with a corroded core and a mantled xenocryst (“ocellus”) of quartz (Qtz), reflecting magma mixing, in a groundmass with elongate laths of zoned plagioclase, hornblende, biotite and interstitial quartz. The mantle on the quartz xenocryst consists of hornblende. Crossed polars; base of photo 8.4 mm.
(6) Restite melting during ascent: Another problem with the solid restite hypothesis is the suggestion of Clemens et al. [1997] and Annen et al. [2006, p. 528] that crystals can melt during ascent of felsic magma, owing to the shallower slopes of P-T mineral stability curves, compared with the steep slopes of magma ascent paths. If so, many or most restite crystals should be removed from felsic magmas by the time they reach temporary storage chambers or final emplacement levels.
(7) Evidence of crystal growth in liquid: The main problem with the solid restite hypothesis is that granitoid minerals mostly show evidence of having crystallized in liquid, namely (a) euhedral crystal shapes, (b) euhedral inclusions arranged zonally (crystallographically) in the host mineral, and (c) zoning patterns (especially oscillatory zoning) reflecting euhedral growth.
Figure 14. Dyke microstructure with K-feldspar oikocryst
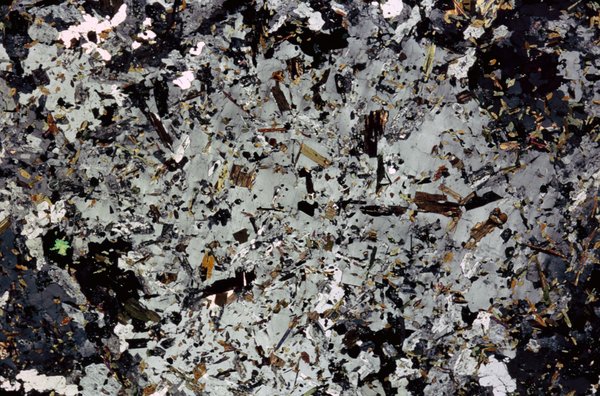
Large poikilitic crystal (oikocryst) of K-feldspar, with numerous, small, predominantly euhedral inclusions of plagioclase, biotite and hornblende, in a synplutonic microgranitoid dyke, Tenaya Lake, Yosemite National Park, California, USA. Though the K-feldspar in this rock grew from few nuclei and developed large grains, the proportion of crystallized other minerals was too large to permit the K-feldspar to grow as a euhedral crystal. This situation is in contrast to that shown in Figure 2, in which the proportion of already crystallized material was low enough and scattered enough for K-feldspar to form large independent crystals by free growth in liquid. Crossed polars; base of photo 8 mm.
(a) Euhedral crystals of quartz and feldspar result from growth in a liquid, for example in felsic volcanic rocks [e.g., Vernon, 1999, 2004]. They are common in porphyritic microgranitoids (Figures 2, 3, 4, 15) and some granitoids (Figure 18), but are rare in metamorphic rocks, except as igneous relics [Vernon, 1986b, 1999, 2004; Vernon and Clarke, 2008, pp. 336-345]. Because fluid along grain boundaries appears to be necessary for the development of euhedral crystals in metamorphic rocks, as discussed by Vernon [2004, pp. 213-216], a reasonable inference is that the absence of crystal faces in metamorphic quartz and feldspar reflects fluid-absent growth in the solid state. In igneous rocks, crystal faces for quartz and feldspar indicate growth in a liquid, and absence of crystal faces is consistent with impingement and interference with other minerals during growth and/or adjustment of boundaries after impingement (Figure 1). Crystal faces for other minerals in granitoids, such as hornblende (Figures 1, 3), biotite (Figure 4), sphene and cordierite, are also consistent with magmatic crystallization.
Figure 15. Dyke microstructure
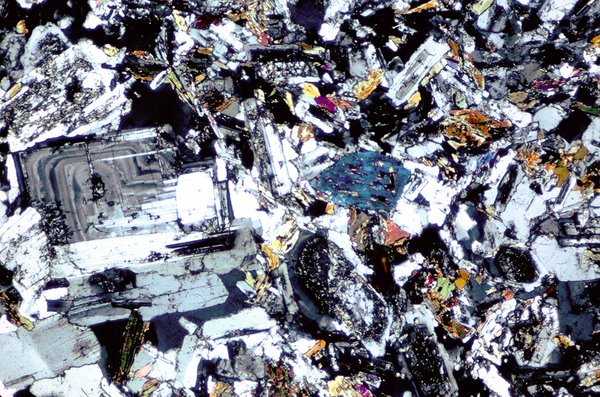
Dyke of porphyritic microgranodiorite, south of Yea, Victoria, Australia, consisting of euhedral plagioclase phenocrysts with oscillatory zoning, in a groundmass of zoned plagioclase laths, hornblende, biotite and interstitial quartz. Crossed polars; base of photo 4 mm.
(b) Zonally arranged mineral inclusions, delineating former euhedral growth surfaces in the host mineral, occur in plagioclase and K-feldspar in some granitoids. Such inclusions are especially common in K-feldspar megacrysts (Figure 18), the inclusions consisting of zoned plagioclase, biotite, hornblende, quartz, apatite, zircon or sphene, as reviewed by Vernon [1986a, pp. 12-15]. Most of the inclusions are arranged in crystallographically controlled zones in the K-feldspar, in such a way that the {001} cleavage in biotite, (010) twin planes in plagioclase, and the longer axes of plagioclase, biotite, hornblende and sphene inclusions are parallel or approximately parallel to the nearest margin, and therefore to former growth faces of the K-feldspar host crystal, as discussed by Hibbard [1965, p. 248], Vance [1969, pp. 19, 21] and Smith [1974, pp. 276-277]. The included minerals are not similarly oriented in the groundmass, indicating they are arranged in this way as a result of incorporation in the megacryst, presumably by rotation to alignment against the K-feldspar growth surfaces. The fact that plagioclase inclusions in K-feldspar have complete zoning patterns indicates that the plagioclase crystals existed independently in the liquid before incorporation, rather than nucleating on the surface of the growing megacryst [Vance, 1969, pp. 19, 21; Vernon, 1986a, p. 14]. Rarely, shells of zonally (crystallographically) arranged inclusions occur in quartz phenocrysts [e.g., Haapala, 1997, p. 1646]. In contrast to this situation, inclusion patterns in metamorphic feldspar are random or delineate ‘inclusion trails’ unrelated to the crystal structure of the host mineral. Therefore, random inclusions or inclusion trails should also occur in any restite or peritectic feldspar grains in granitoids, but these features are typically not observed. In any event, grains with such features would be difficult to identify as restite or xenocrysts [e.g., Vernon, 2007].
Figure 16. Microgranitoid enclave in dacite
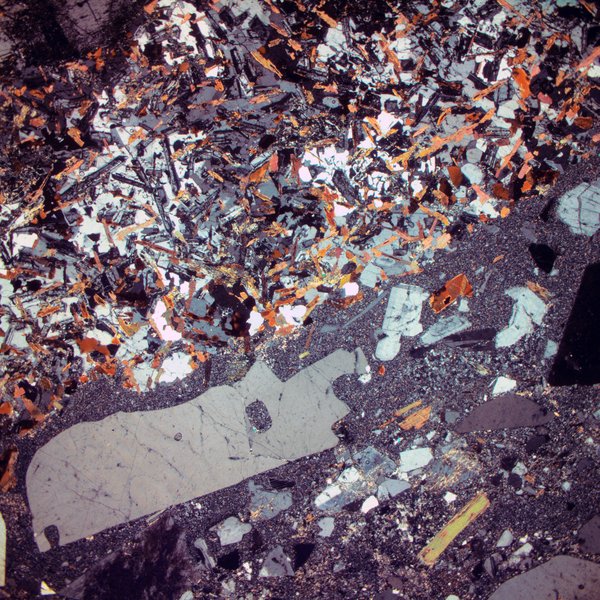
Microgranitoid (microtonalite) enclave (top-left half of photo) in dacitic ignimbrite consisting of phenocrystic fragments of embayed quartz, zoned plagioclase and biotite, Violet Town Volcanics, Victoria, Australia. The enclave has an igneous microstructure, with elongate grains of plagioclase and biotite and interstitial, poikilitic quartz. Crossed polars; base of photo 7 mm.
Figure 17. Miarole in microgranitoid enclave
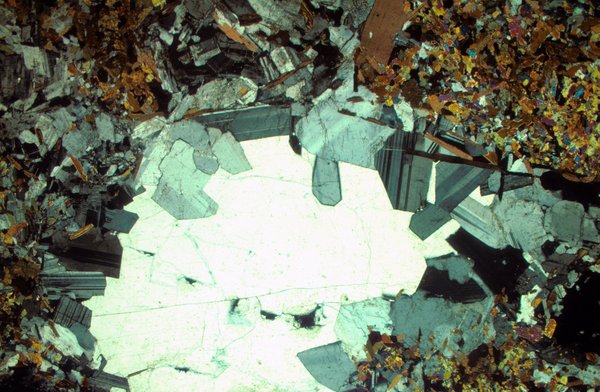
Miarole (miarolitic cavity) characterized by euhedral plagioclase crystals projecting into quartz. The former cavity was formed by escape of gas from the hybrid enclave magma, followed by cooling sufficiently rapid to avoid collapse of the bubble, permitting later quartz to crystallize. Crossed polars; base of photo 7 mm.
Figure 18. Crystallographically arranged inclusions in K-feldspar
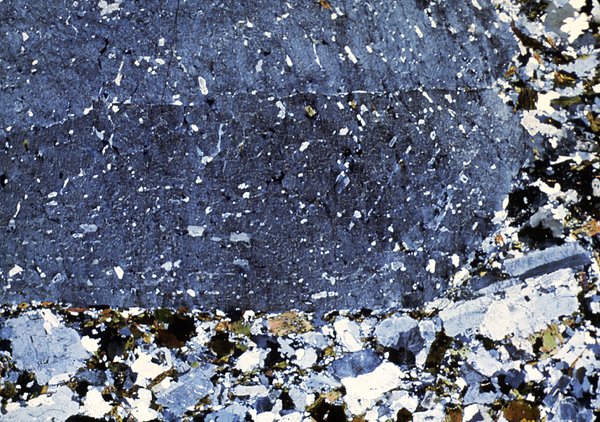
Part of a euhedral megacryst of K-feldspar with zonally arranged inclusions of plagioclase, in the Wuluuman Granite, Wellington, New South Wales, Australia. Parallel to the rows of inclusions are faint darker and lighter bands that represent oscillatory zoning. Crossed polars, base of photo 22 mm. Photo by Bill D’Arcy. From Vernon (2004, figure 3.12).
(c) Oscillatory zoning in granitoid minerals is a strong indicator of magmatic growth, because it is invariably inferred to result from growth in liquid [e.g., Vance, 1962; Boone, 1962; Boesen, 1964; Hibbard, 1965, p. 253; Bottinga et al., 1966; Wiebe, 1968; Boyd and Smith, 1971; Zeck, 1972; Gibb, 1973; Smith, 1974, pp. 212-214; Downes, 1974; Sibley et al., 1976; Haase et al., 1980; Mehnert and Büsch, 1981; Barton et al., 1982; Eriksson, 1985; Clark et al., 1986; Roycroft, 1989, 1991; Halden and Hawthorne, 1993; Jones and Papike, 1993; Shore and Fowler, 1996; Hattori and Sato, 1996; Fowler et al., 2002; Bachmann and Dungan, 2002; Dempster et al., 2003; Vernon, 2004, pp. 140-146, 262-268; Erdmann et al., 2005, 2009, p. 497; Sato et al., 2005; Féménias et al., 2006; Wiebe et al., 2007, p. 449; Tsune and Toramaru, 2007; Burger et al., 2009; Dziggel et al., 2009].
Figure 19. Oscillatory zoning in K-feldspar megacryst
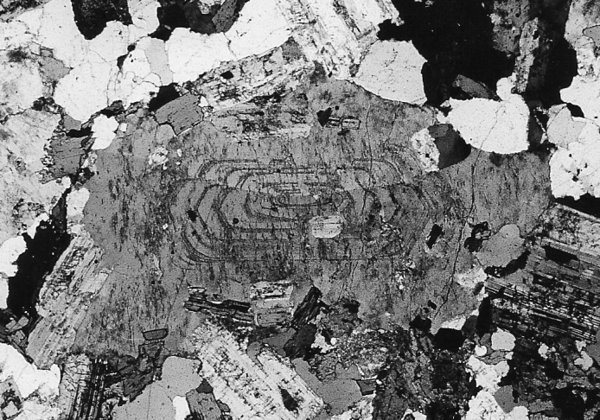
Megacryst of K-feldspar with prominent oscillatory zoning and simple twinning in the Walcha Road pluton, New England Batholith, near Walcha, New South Wales, Australia. Crossed polars; base of photo 7 mm. Photo by Bill D’Arcy.
In granitoids, oscillatory zoning occurs in plagioclase (Figures 1, 5), K-feldspar (Figures 18, 19) quartz (Figure 6), hornblende (Figure 20), cordierite [Erdmann et al., 2009, fig. 9d], sphene, zircon, apatite [Dempster et al., 2003] and allanite [e.g., Shaw & Flood, 2009, fig. 2b], as revealed by optical and/or scanning electron microscope (SEM) techniques. Oscillatory zoning is especially common in plagioclase [e.g., Vance, 1962; Hibbard, 1965; Bottinga et al., 1966; Wiebe, 1968; Smith, 1974; Sibley et al., 1976; Haase et al., 1980; Vernon, 2004; Tsune and Toramaru, 2007] and K-feldspar [Oen, 1960; Boone, 1962; Bateman et al., 1963, p. D15; Vaniman, 1978; McTaggart, 1979; Michael, 1981; Phillips et al., 1981, p. 362; Mehnert and Büsch, 1981; Brigham, 1984; Vernon, 1986a] in granitoids. Oscillatory zoning in quartz in high-level granitoids (Figure 6) has been revealed by cathodoluminescence [e.g., D'Lemos et al., 1997; Seyedolali et al., 1997; Watt et al., 1997; Müller et al., 2000, 2002a, 2002b, 2005; Peppard et al., 2001; Wiebe et al., 2007; Larsen et al., 2009], but whether or not it commonly occurs in deeper granitoids is uncertain.
Oscillatory zoning in euhedral hornblende phenocrysts in dacite (derived from granodioritic magma) of the Fish Canyon Tuff, Colorado, USA, has been described and illustrated by Bachmann and Dungan [2002], and oscillatory zoning in euhedral hornblende phenocrysts in dacite of the Unzen Volcano, Japan, has been described and illustrated by Sato et al. [2005, figure 1, pp. 340-341], the zoning showing corrosion breaks that correspond to similar discontinuities in oscillatory zoning in plagioclase phenocrysts, caused by magma mixing. Oscillatory zoning also occurs in euhedral hornblende phenocrysts in andesite erupted at the Soufrière Hills Volcano, Montserrat [Rutherford and Devine, 2003, figures 1c, 1d], an andesite dyke, Motru Dyke Swarm, Romania [Féménias et al., 2006] and a porphyritic microgranodiorite dyke from Hartley, New South Wales, Australia (Figure 3). All these zoned hornblende crystals crystallized from felsic to intermediate magma at depth, before volcanic eruption or dyke emplacement, and so indicate that oscillatory zoned hornblende can form in the plutonic environment, at least at relatively high crustal levels. A detailed search for oscillatory zoning in hornblende in a range of granitoid plutons is needed, to determine the extent of its development.
Oscillatory zoning occurs in muscovite in some peraluminous granites [Roycroft, 1989, 1991], but does not appear to have been previously reported in biotite. However, SEM images of Ba oscillatory zoning in biotite in the Half Dome Granodiorite, Tuolumne Batholith, California, USA, have been obtained by Joachim Krause [personal communication], and so zoned biotite in granitoids is worth further investigation.
Oscillatory zoning in granitoids is strong evidence that granitic minerals crystallize from liquid, because the zoning preserves former euhedral shapes developed as the crystal grows, constituting a ‘growth stratigraphy’ [Wiebe, 1968]. The regular oscillatory pattern can be locally interrupted and truncated by resorption events, after which oscillatory growth resumes [e.g., Shore and Fowler, 1996; Roycroft, 1991]. The zoning patterns can record elimination of fastest growing faces, temporary faces, temporary face widening, and disruption to patterns caused by inclusions [e.g., Roycroft, 1991, figure 2], indicating that the zoning is a primary growth feature, not one formed by subsolidus alteration processes.
Figure 20. Oscillatory zoning in hornblende
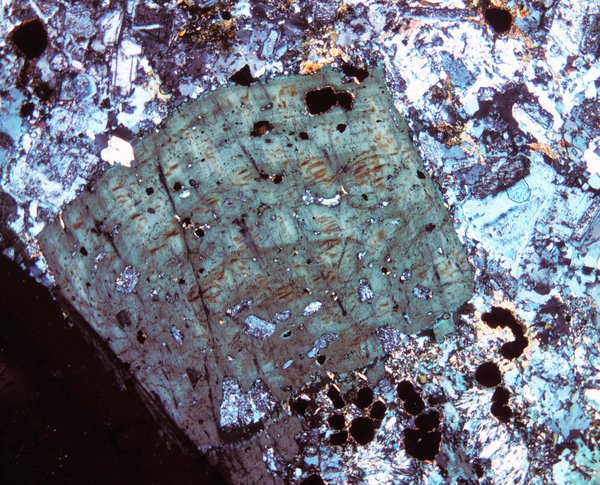
Oscillatory zoning in hornblende in the Ravenswood Granodiorite, north Queensland, Australia. Crossed polars; base of photo 3.5 mm.
The euhedral oscillatory zoning patterns commonly extend right to the centre of the crystal (Figures 1, 5, 6), reflecting crystallization from a liquid for the crystal’s entire growth history, except for late overgrowths that interpenetrate with neighbouring minerals (Figures 1, 5), and therefore rule out a restite origin. In contrast, oscillatory zoning in metamorphic minerals is uncommon, apart from occurrences in skarns and hydrothermal veins [e.g., Shore and Fowler, 1996, p. 1117]. Oscillatory zoning in metamorphic garnet has been reported, as summarized by Vernon [2004, pp. 265-266], but is typically less sharply defined than in igneous minerals, and is generally attributed to open-system growth conditions involving changes in fluid composition [Jamtveit, 1991; Jamtveit and Andersen, 1992; Jamtveit et al., 1993; Boundy et al., 2002; Clechenko and Valley, 2003; Dziggel et al., 2009]. Moreover, the zoning patterns typically reflect successive crystal faces developed during growth [e.g., Schumacher et al., 1999; Vernon, 2004, pp. 263-265], indicating growth in at least a small amount of fluid [e.g., Kingery, 1960; Budworth, 1970; Sunagawa, 1974, 1977; Sunagawa et al., 1974; Tomura et al., 1979; Urai, 1983a, 1983b; Urai et al., 1986; Rakovan and Jaszczak, 2000; Vernon, 2004, pp. 213-216, 263].
The well defined oscillatory zoning that is common in metamorphic grossular-andradite garnet invariably reflects growth in fluid-filled open cavities [Vernon, 2004, p. 263], as illustrated by Clechenko and Valley [2003, figs 2c, 2d], Intayot et al. [2007, p. 69, figures 2, 3] and Dziggel et al. [2009, fig. 6]. Oscillatory zoning in prehnite and clinopyroxene precipitated from hydrothermal fluids in cavities has been observed by Yardley et al. [1991], and is well known in many other minerals that crystallize in open spaces from fluids [Shore and Fowler, 1996, table 1]. All these examples show a ‘growth stratigraphy’ [e.g., Wiebe, 1968] outlined by former crystal faces preserved in the zoning patterns.
Generally, metamorphism and hydrothermal alteration remove pre-existing oscillatory zoning. For example, Long and Luth [1986] noted that hydrothermally altered parts of the Puntiagudo granite porphyry, New Mexico, USA, contain homogenized Ba in formerly zoned K-feldspar megacrysts. Therefore, it is reasonable to infer that any putative restite in granitoids would not show oscillatory zoning. The contrast between replacement and primary magmatic hornblende is well shown by Fe and Al X-ray maps produced by Joachim Krause (personal communication); the replacement hornblende is unzoned, whereas the outer magmatically precipitated hornblende shows an oscillatory zoning pattern.
General inference about restite in granitoids: The implication of the foregoing discussion of predominantly microstructural evidence is that most minerals in granitoids crystallize from liquid, rather than being solids inherited from source rocks. Most fragmental foreign grains are difficult or impossible to distinguish on microstructural grounds, and so could be xenocrysts, restite (including peritectic minerals) or their modified equivalents.
However, absence of microstructural evidence of solid restite in granitoids does not preclude the presence of potential or former restite chemical components dissolved in the liquid. For example, restite components could enter the liquid if solid restite crystals were dissolved during ascent [e.g., Annen et al., 2006, p. 532], or if they reacted with the liquid to produce new minerals or compositional variants of the original minerals. When such components are dissolved, they end up in magmatic crystals, and so cannot be identified as such. Higher-temperature components (which would otherwise occur in restite) also could enter the liquid in the source, owing to relatively high melting temperatures, so that different magma batches in a collection zone could contain different proportions of higher-temperature components, depending on the local melting temperature in those parts of the source region from which the batches were extracted, while remaining entirely magmatic. High-temperature melting would be especially likely in ‘deep hot zones’, which is where many large granitic magma batches are probably generated [e.g., Annen et al., 2006, 2008; Vernon, 2007].
The main conclusion is that most minerals in granitoids crystallize from liquid, regardless of how their chemical components enter the liquid, and that unambiguous solid restite or peritectic grains and aggregates from the source are uncommon, apart from modified garnet grains in some peraluminous granitoids and inherited zircon crystals or cores.