Limitations of microstructural interpretation
Some petrologists have suggested that granitoid microstructures should not be used for petrogenetic interpretations because they are unreliable. This reservation has been clearly expressed by Means and Park [1994, p. 323], who wrote: “in plutonic igneous rocks …, it is not even entirely clear which textural features are produced during magmatic crystallization and which represent later, solid-state adjustments. On the contrary, I contend that it is generally possible to make such a distinction, except for minor irregularities at the margins of some grains (Figure 1), which could be due either to impingement of two minerals with weakly anisotropic crystal structures or to minor grain-boundary adjustment after impingement. Detailed evidence for distinguishing between magmatic and solid-state microstructures has been presented by Vernon (1999, 2000, 2004) and Vernon and Paterson (2008c), and will be further discussed in the evaluation of hypothesis 3.
Early stages of crystallization: A limitation of microstructural interpretation is that a rock’s structure is the end-product of a possibly complex history (as is a rock’s chemical analysis), the early stages of which may not be well preserved [e.g., Means and Park, 1994; Johnson and Glazner, 2010]. Inferences about early crystallization processes in magmas have been made from observations of (a) synkinematic crystallization experiments using analogue compounds and (b) glassy volcanic rocks. However, such observations can be misleading for the interpretation of granitoid microstructures. For example, low-temperature, synkinematic, analogue experiments by Means and Park [1994] revealed several processes that could conceivably occur in magmas, such as coarsening of graphic intergrowths to form independent crystals. Graphic intergrowths occur in some high-level granitoids, but evidence of coarsening to form independent grains is not observed. Solid-state adjustment of symplectites, forming globular to polygonal grains, occurs in some metamorphic rocks [e.g., Vernon, 2004, pp. 256-261; figure 4.65]; however, this situation is rarely, if ever, duplicated in granitoid plutons, because it would have to involve early undercooling to form the intergrowth, followed by prolonged heating to coarsen the aggregate. In contrast, the entire crystallization history of most granitic magma in plutons is likely occur at relatively small degrees of undercooling, owing to slow cooling rates, leading to relatively high proportions of crystal faces and euhedral zoning patterns in the resulting granitoids (Figure 1). The contrasting effects of strong undercooling are well shown by rapidly cooled pegmatites and granophyres, which are characterized by graphic intergrowths [London, 1996, 2005, 2008; Vernon, 2004, figure 3.55; pp. 115-119]. Some high-level granites show normal quartz and feldspar phenocrysts merging into graphic intergrowths, owing to a change in conditions from slow to rapid cooling, with a consequent increase in the degree of undercooling.
Figure 1. Grain shapes and oscillatory zoning in granodiorite
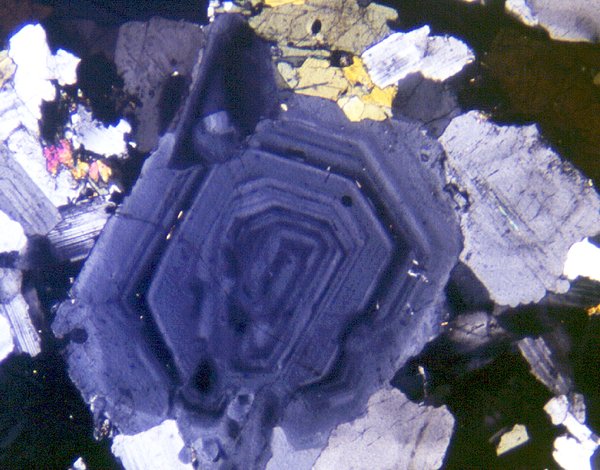
(A) Granodiorite, Tenaya Lake, Sierra Nevada Batholith, California, USA, showing plagioclase with complete oscillatory zoning patterns, indicating that solid-state grain-shape changes during cooling were negligible. Some plagioclase boundaries against quartz and other plagioclase grains (for example, at the right of the image) are planar and appear to be crystal faces, reflecting unimpeded growth in liquid. In contrast, other plagioclase/plagioclase and plagioclase/quartz boundaries are irregular, reflecting mutual interference during simultaneous growth, minor subsolidus grain-boundary adjustment, or both. The hornblende crystal at the top of the image has crystal faces against quartz, reflecting unimpeded growth in a liquid, and also shows a simple twin, which probably indicates growth from a twinned nucleus. Crossed polars; base of photo 4.5 mm.
(B) Oscillatory growth zoning in plagioclase in tonalite, San José pluton, Baja California, México. The zoning developed through the whole growing history of the crystal, and the pattern reveals successive stages in the crystal’s shape. The crystal boundaries are partly euhedral and partly indented, owing to impingement with adjacent grains during growth or minor deformation. Crossed polars; base of photo 2 mm. From Vernon (2004, fig. 3.45).
Similarly, inferences made from observations of phenocryst-free glassy volcanic rocks need not be relevant for coarse-grained compositional equivalents, because the volcanic rocks represent very rapid crystallization at conditions of strong undercooling that are unrealistic for magmas at depth. On the other hand, rapidly cooled rocks that previously had undergone some crystallization at low degrees of undercooling do preserve a ‘snapshot’ of the mineral assemblage and microstructure at the instant of chilling (Figures 2, 3), and so are relevant to the interpretation of granitoid early crystallization history (see evaluation of hypothesis 2).
Johnson and Glazner [2010] wrote: “only by textural coarsening can coarse-grained plutonic textures form from finer-grained equivalents.” This statement appears to assume that coarse-grained plutonic rocks initially consist of fine-grained crystal aggregates that coarsen by solid-state grain growth. On the contrary, crystallization in most granitic magmas probably initiates as scattered independent crystals, owing to low nucleation rates at small degrees of undercooling [e.g., Vernon, 2004, pp. 46-54; figures 3.2, 3.6], after which the small crystals could simply grow larger while new ones nucleate. This situation is illustrated by Figures 2 and 3, which show many separate crystals, with or without some glomeroporphyritic aggregates, at a relatively early stages of crystallization. As part of this process, a crystal dissolution/coarsening process (‘Ostwald ripening’) could apply to the very early stages of crystallization, when interfacial free energy differences between minute particles can drive diffusion and so contribute to the formation of viable nuclei by eliminating smaller particles that are close enough for diffusion to be effective [e.g., Vernon, 2004, pp. 51-52].
Figure 2. Unimpeded growth of K-feldspar megacryst in liquid
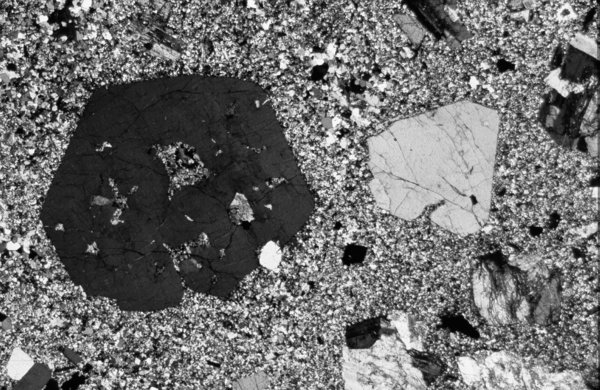
(A) Porphyritic microgranodiorite, Yetholme, New South Wales, Australia, showing a twinned megacryst of K-feldspar (Kfs), together with much smaller phenocrysts of plagioclase (Pl), quartz (Qtz) and biotite (Bt), all set in a very fine-grained quartz-feldspar groundmass. All phenocrysts show a high proportion of crystal faces, owing to free growth in liquid. Rapid cooling has preserved evidence of growth of the K-feldspar megacrysts in liquid. The K-feldspar grew relatively rapidly from few nuclei. Glomeroporphyritic aggregates (especially of plagioclase) are more common in crystal-rich parts of the rock, which is consistent with fortuitous impingement, but does not exclude heterogeneous nucleation. The quartz phenocrysts have embayments, cross-sections of which appear as rounded to irregular patches (pseudo-inclusions) of groundmass. Crossed polars; base of photo 29 mm. Photo by Bill D’Arcy.
(B) Same rock as in (A), showing independent euhedral phenocrysts of quartz and plagioclase in an abundant fine-grained groundmass. Crossed polars; base of photo 13 mm. Photo by Bill D’Arcy.
Figure 3. Separate euhedral phenocrysts
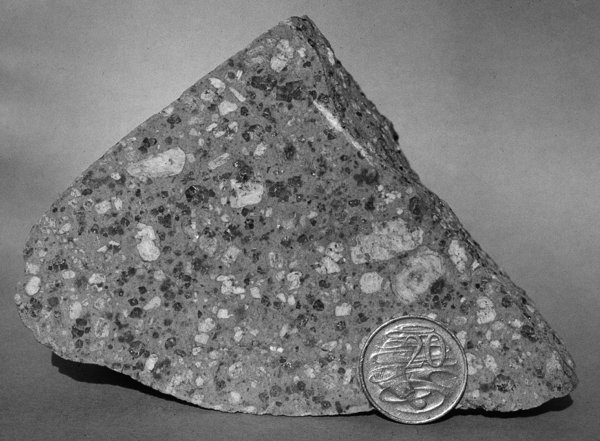
(A) Separate, euhedral megacrysts of K-feldspar in a granitoid, suggesting independent (possibly homogeneous) nucleation in abundant liquid. Some of the megacrysts show oscillatory zoning or crystallographically arranged zones of inclusions. Sawn slab; base of photo 25 cm.
(B) Predominantly separate euhedral phenocrysts of plagioclase and quartz in a dacite. Sawn slab; coin diameter 2.8 cm.
Jerram et al. [2003] reviewed the evidence for crystal clustering in igneous rocks, implying that this is a basic process in the development of magmatic rock structure, involving either heterogeneous nucleation or fortuitous coalescence. Clustering is an inevitable result of crystal accumulation by either mechanical processes or removal of interstitial liquid [e.g., Vernon & Collins, 2011]. For example, relatively coarse-grained (glomeroporphyritic) aggregates or ‘clots’, generally interpreted as being of cumulate origin, are common in calc-alkaline volcanic rocks [e.g., Flood et al., 1977; Garcia & Jacobson, 1979; Scarfe & Fujii, 1987; Renzulli & Santi, 1997; Gençalioğlu Kuşcu & Floyd, 2001]. The aggregates appear to represent precipitates of a parental magma, generally at an earlier stage of development than that represented by the phenocrysts of the host magma.
Jerram et al. [2003, p. 2049] stated, without explanation, that “in more slowly cooled intrusive bodies, clustering would be expected to occur very early in the crystallization history of the magma, although it may occur over longer time-scales because of slower growth rates in the plutonic environment.” However, the situation represented by Figures 2 and 3 suggests that clustering is relatively uncommon in the early stages of crystallization in slowly cooled felsic magmas. Instead, crystals generally appear mainly to have nucleated separately, though local clustering can occur. This situation is shown by many felsic and intermediate, porphyritic, shallow intrusive and volcanic rocks (non-pyroclastic), which are characterized by mainly dispersed, euhedral phenocrysts [e.g., Vernon, 2004, figures 3.5, 3.7, 3.8, 3.9, 3.13; McDonnell et al., 2004, figure 2]. For example, O’Donnell and Hogan [2004] and Hogan and O’Donnell [2008] described a rhyolite dyke carrying euhedral to subhedral phenocrysts of quartz (25%) and perthitic K-feldspar (75%) in a fine-grained quartz-feldspar groundmass, most of the phenocrysts being independent, some occurring in glomeroporphyritc aggregates. Moreover, studies of over 6000 zircon crystals and over 3000 quartz crystals in felsic pumice (reflecting instantaneous conditions in the granitic parent magma) have revealed only two zircon aggregates and five quartz aggregates, confirming that independent crystallization of these minerals in felsic melts is typical [Bindeman, 2003, p. 367]. In view of this situation, most of the clustering in granitoids is probably due to fortuitous impingement, rather than heterogeneous nucleation, and so would be favoured by higher crystal concentrations. Mock & Jerram [2005, p. 1538] suggested that the relatively high yield strength of felsic liquids could allow phenocrysts to be suspended without interconnection at higher proportions of crystals than more mafic liquids. Under conditions of slow cooling in plutons, crystal aggregates are formed by liquid movement, causing fortuitous impingement of grown crystals, forming chain structures [see Vernon, 2004, pp. 109-115 for a summary] or igneous cumulates, including both cumulates formed by physical accumulation and cumulates formed by removal of interstitial liquid [e.g., Vernon and Collins, 2011].
Most published examples of clustered nucleation occur in strongly undercooled rocks, such as basalts and komatiites [e.g., Kirkpatrick, 1977, 1981; Jerram and Cheadle, 2000; Jerram et al., 2003], for which nucleation is forced to occur heterogeneously [e.g., Vernon, 2004, figures 3.4, 3.50]. Hersum and Marsh [2007, p. 250, figure 3] suggested that aggregated small crystals in basaltic lava lakes grow into larger ones by consuming still smaller crystals, in a process of grain-boundary migration, by which grains with lower total surface free energy (normally the larger crystals) survive and grow. Hersum and Marsh [2007] wrote that “the process may also continue, given the proper opportunities, at high crystallinities where quenching is uncommon” and that “the kinetics of this process of grain annexation, which is, in effect, a form of high-temperature annealing, are so rapid that little evidence of such growth is left in the final rock record.” Mock and Jerram [2005, p. 1537] also emphasized “crystal growth by annexation of small crystals into large ones (after abutment of crystals) by grain boundary diffusion, producing optically continuous large crystals.”
However, there is no clear evidence connecting this inferred behaviour in lava lakes, where cooling is rapid at large degrees of undercooling (leading to heterogeneous nucleation and crystal clustering), with crystallization of coarse-grained gabbros, where temperatures would be higher for longer periods, undercooling would be minimal, and consequently nucleation rates would be relatively low, resulting in fewer, more widely separated nuclei that would grow into larger independent crystals in liquid. Grain-shape modification could occur only after impingement of these large crystals, evidence of which is found in some gabbros, anorthosites and peridotites (see section dealing with hypothesis 3). However, evidence of such modification is generally absent from granitoids, as shown in Figures 1 and 4 (see section dealing with hypothesis 3).
Another argument against the grain-boundary migration coarsening process proposed by Hersum and Marsh [2007] and Johnson and Glazner [2010] is that the abundance of crystals with oscillatory growth zoning extending right to the centre in granitoids (Figures 1, 2, 5, 6) eliminates the possibility of grain coarsening by grain-boundary movement after very small crystals have developed. Although such zoning patterns can be disrupted by changes in magmatic conditions, they typically outline former crystal growth surfaces [e.g., Wiebe, 1968], reflecting crystallization in liquid. The growth zones generally show no evidence of major partial removal at crystal edges by migrating grain boundaries after solidification, except for uncommon examples of contact melting [e.g., Vernon et al., 2004; Vernon, 2004, pp. 462-463], as discussed later.
Figure 4. Crystal faces and local poikilitic structure
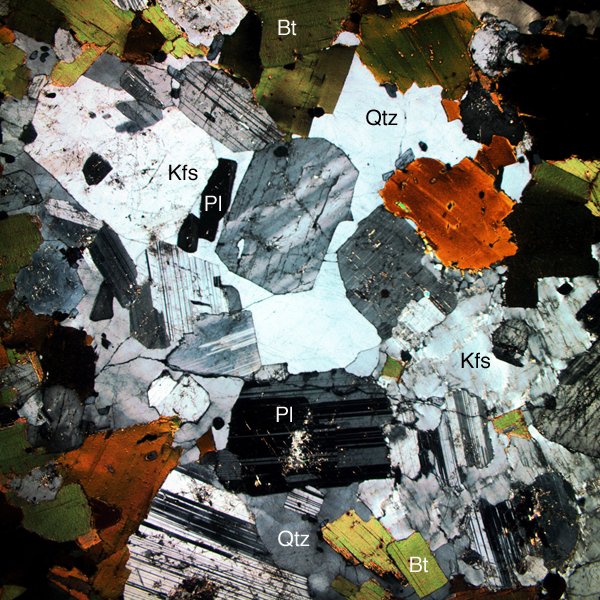
Plagioclase (Pl) and biotite (Bt) with crystal faces against quartz (Qtz) and K-feldspar (Kfs), as well as local poikilitc microstructure consisting of small crystals of plagioclase enclosed in quartz and K-feldspar, Kameruka Granodiorite, Bega Batholith, south-eastern Australia. A possible interpretation is that the small plagioclase crystals represent relatively late crystals that grew in interstitial liquid at the same time as more rapid growth from fewer nuclei of quartz and K-feldspar. Crossed polars; base of photo 7 mm.
The concept of grain coarsening conceivably could be used to explain relatively small inclusions, compared with the sizes of other grains of the same mineral in some granitoids (Figure 4), by implying that originally all crystals of the included minerals are small (when some of them are incorporated in the host crystal), after which the non-included crystals grow at the expense of others that dissolve. However, this process (“Ostwald ripening”) is limited by diffusion rates [e.g., Carlson, 1999, 2000] and grainsize. For surface free energy to dominate the volume chemical free energy to the extent that it drives dissolution, the particles must be very small, in the micrometre or smaller size range (Jackson, 1967; Martin and Doherty, 1976; Baronnet, 1982; Lasaga, 1988, p. 150; Vernon, 2004, p. 52). Therefore, the process would only apply in the every early (post-nucleation) stages. Experiments have shown that Ostwald ripening is negligible for millimetre-size quartz crystals in rhyolitic magma [Cabane et al., 2001] and this has been confirmed by CSD studies of quartz crystals in rhyolitic pumice [Bindeman, 2003, p. 369]. The alternative idea, that the coarsening of non-included grains occurs in the solid state (grain growth) is theoretically viable, but should result in metamorphic-like aggregates, which are generally not observed in granitoids (see section dealing with hypothesis 3).
In view of these considerations, a preferable explanation of inclusions that are smaller than surrounding grains of the same mineral is that the inclusions represent crystals that nucleate in interstitial liquid during the later stages of growth of the larger crystals, as nucleation rates begin to increase and growth rates decrease. Theoretically, large and small crystals should coexist, because the crystallization of liquids involves continuous nucleation and growth [Hersum and Marsh, 2007, p. 248]. So, even if a magma is held at small degrees of undercooling for most of its cooling history, the last remaining liquid would be expected to nucleate new crystals, with the result that some smaller crystals should always be present. However, they would probably be scattered sparsely through an otherwise coarse-grained aggregate, and so could be hard to detect. On the other hand, detailed CSD analysis of many quartz and zircon crystals in rhyolitic pumice (representing magma practically instantaneously extracted from a felsic pluton) by Bindeman [2003] shows a deficiency of smallest crystals, which suggests that nucleation in slowly cooling felsic magmas does not occur homogeneously, but occurs by forming layers on existing crystals. This could apply to feldspar as well [Bindeman, 2003]. On the other hand, similar under representation of small sizes in quartz and feldspar CSD curves for a rhyolite dyke have been attributed to resporption during ascent decompression by O’Donnell and Hogan [2004] and Hogan and O’Donnell [2008]. The relative uniform dispersal of phenocrysts in many felsic rocks (Figures 2, 3) is consistent with homogeneous nucleation for the formation of the intial crystals, on which later growth can occur, though clear evidence of the initial nucleation process is unavailable. The large grains of poikilitic quartz and K-feldspar illustrated in Figure 4 could have grown at the same time as the fine-grained plagioclase inclusions, but from few nuclei. This interpretation, if correct, emphasizes the complications caused by variations in nucleation rate between different minerals in granitoids (see section on order of crystallization, below).
Figure 5. Early crystallization ‘snapshot’
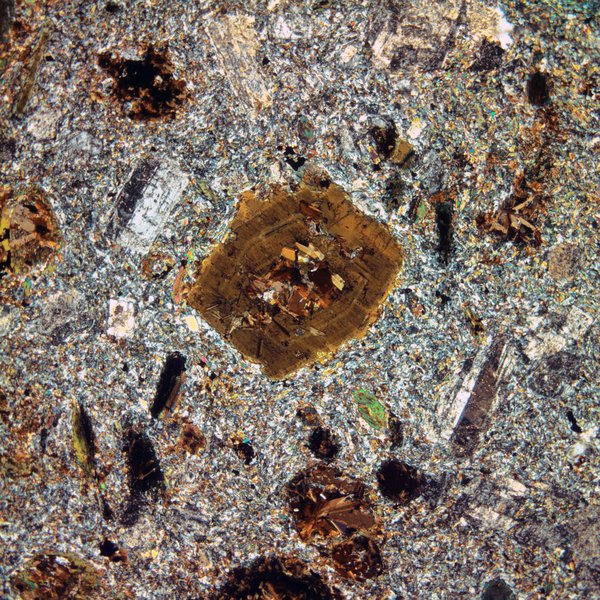
Porphyritic microgranodiorite dyke, Hartley, New South Wales, Australia, showing evidence of the earlier stages of crystallization, in the form of isolated, euhedral plagioclase and brown hornblende phenocrysts with oscillatory zoning patterns that reflect the growth histories of the crystals. Much of the hornblende has been replaced by aggregates of biotite, inferred to be of subsolidus origin. The central hornblende phenocryst shows abunbdant oscillatory zoning and only minor replacement by late biotite flakes. Crossed polars; base of photo 7 mm.
Spasmodic events: Intermittent re-heating episodes, commonly associated with magma mixing and/or convection, are responsible for zoning discontinuities and mantled crystals, which are common in granitoids and equivalent volcanic rocks [e.g., Wiebe, 1968; Davidson et al., 1988, 2007, Charlier et al., 2005; Jerram and Davidson, 2007], as well as coexistence of crystals with different zoning patterns [e.g., Collins et al., 2006; Vernon and Paterson, 2008a]. Mechanical accumulation of crystals and/or passive accumulation through loss of interstitial liquid are also prominent processes in granitic magmas [e.g., Paterson et al., 2005; Collins et al., 2006; Vernon and Paterson, 2008a]. Spasmodic re-heating of crystals in response to mafic magma replenishments and/or convection can cause dissolution of small crystals and transfer of dissolved components to nearby larger crystals [e.g., Higgins and Roberge, 2003]. However, though all these processes enrich and complicate the crystallization history, they do not alter the overall magmatic nature of granitoids.
Figure 6. Oscillatory zoning in quartz
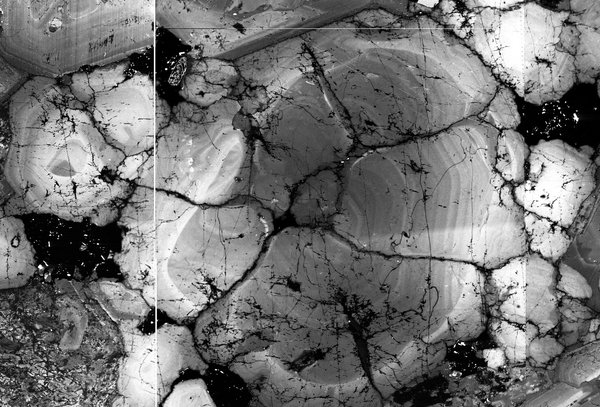
Aggregate of quartz phenocrysts in the Vinalhaven pluton, Maine, USA, showing euhedral concentric oscillatory Ti zoning revealed by SEM cathodoluminescence, reflecting former growth habits. The zoning in the outer part of the largest crystal has been truncated and rimmed by new brighter zones richer in Ti, the truncation having been caused by dissolution in response to replenishment by more mafic magma. The dissolution was followed by precipitation of Ti-enriched quartz from the heated felsic magma (Wiebe et al., 2007). Mosaic of cathodoluminescence images; largest quartz crystal 4.2 mm across. Photo by Bob Wiebe.
Figure 7. Contact melting
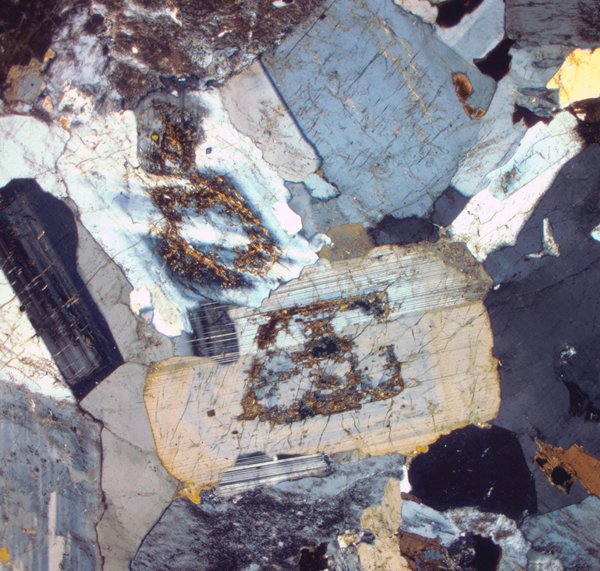
Irregular interface, consistent with contact melting, between two zoned plagioclase grains, each of which has a complete core (outlined by preferentially altered zones), reflecting a period of independent growth in liquid before impingement. Wards Mistake Adamellite, New England Batholith, New South Wales, Australia. Crossed polars; base of photo 3.7 mm.
Order of crystallization: A problem that generally cannot be solved by microstructural evidence, is the inference of an order of nucleation of minerals in granitoids, as argued by Flood and Vernon [1988] and Vernon [1996, 2004], who showed that inclusion and moulding relationships are unreliable, and that the only structures reliably indicating an order of nucleation are mantles or corona structures formed by discontinuous reactions, such as hornblende rims on pyroxene (Figure 8). An additional complication is suggested by the analogue experiments of Means and Park [1994], which revealed migration of grain boundaries during cooling. Means and Park [1994, p. 326] emphasized that if melt-present grain- and phase-boundary migration are important, caution must be exercised when “classically” interpreting the order of crystallization from microstructural observations. As an example of “classically” interpreted orders of crystallization, they cited Flood and Vernon [1988], which was unfortunate, because Flood and Vernon [1988] took great pains to point out that the classical approach is invalid, and that moulding and impingement relationships should not be used to make inferences about an order of crystallization. This warning was re-emphasized by Vernon [2004, pp. 102-108].
Figure 8. Discontinuous reaction relationship
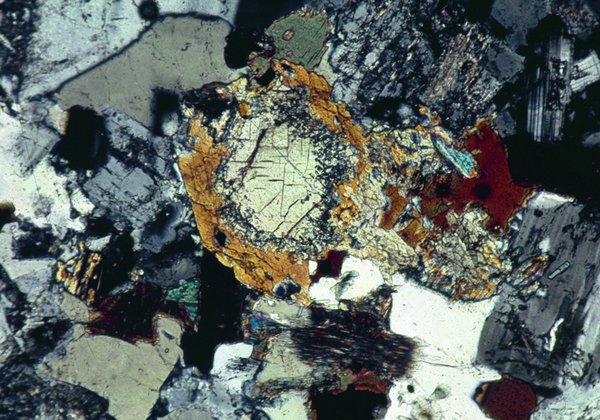
Relic of pyroxene mantled and partly replaced by hornblende (a discontinuous magmatic reaction relationship) in granodiorite, Uralla, New South Wales, Australia. This is one of the few microstructural relationships indicative of an order of nucleation. Crossed polars; base of photo 4.4 mm.
General inference: The foregoing discussion indicates that the main limitations to microstructural interpretation are that (1) evidence of the earliest stages of crystallization is generally not available, and (2) microstructural evidence should not be used to infer an order of nucleation, except for corona structures. However, provided these limitations are kept in mind, microstructural evidence can be applied to petrogenetic problems of granitoids, for example, problems posed by the following three hypotheses.