What we imagine: the geodynamic evolution
The pieces of information presented in the above sections are integrated in a geodynamic scenario dominated by the convergence of Africa against Europe. In the Italian area, three subduction zones active during the Cenozoic consumed both oceanic and continental lithosphere (Doglioni and Carminati, 2002): the Alpine (with the European Plate subducting below the Adriatic micro-plate), the Apenninic (with the nearly completely consumed Ionian/Mesogean oceanic lithosphere and the Adriatic micro-plate subducting westward beneath the European Plate and characterised by a strong “eastward” radial retreat of the subduction hinge), and the Dinarides (with the Adriatic micro-plate subducting north-eastward under the European Plate). The third subduction, that is marginal in the Italian region, is no longer treated in this review. The following geodynamic reconstruction is shown in the movie associated with this paper, downloadable from the Journal of Virtual Explorer repository system.
The movie: some information and caveats
Although a full and detailed description of the evolution of the entire western and central Mediterranean region is beyond the scope of this work, the map-view movie attached to this paper includes also features external to the Italian area (e.g., Pyrenees, Dinarides, Betics, Rif, Maghrebides, Carpathians, Pannonian Basin), not treated in this work. This was done in order to place the geodynamic evolution of the Italian region in a more regional context. For more details on the evolution of the Mediterranean region and for alternative views, the interested reader is referred to previous reviews of the geology and geodynamics of the central and western Mediterranean area (e.g., Faccenna et al., 1997. Carminati et al., 1998; Gueguen et al., 1998; Wortel and Spakman, 2000; Tari, 2002; Csontos and Voros, 2004; Carminati and Doglioni, 2005; Rosenbaum and Lister, 2004; Mauffret, 2007; Chalouan et al., 2008).
The movie that is here presented is intended to be as rigorous as possible. However, the scale of the representation and the complexity of the evolution of the region are such that some features had to be represented in a schematic way. For example, the normal faults associated with the opening of the back-arc basins are not to be taken as representative of real faults but indicate the location where normal faulting occurred. Moreover, we have to admit the following uncertainties and under-constraints in the paleogeographic reconstructions. A general (and easily understandable) rule is that the uncertainties are larger for older time frames. In the 50-40 Ma frames of the movie, the geometry of the Liguro-Piedmont-Penninic ocean is largely speculative.
Another poorly constrained point is the contact between the Betics and Rif mountain belts, that, in the movie is idealized with a straight line. As concerns the age of the igneous activity, most of the geochronological data are incomplete and scattered (no systematic cover exists). Most of the isotopic ages are based on old (produced during ‘70s-‘80s) K-Ar ages and only very few are based on detailed 40Ar/39Ar ages. Moreover, as in the Tyrrhenian Sea submerged igneous rocks, only the most recent activity has been dredged/cored. This means that the ages should be considered only as the youngest limit. No one knows exactly when igneous activity started in these and other igneous districts.
In order to clarify the evolution of the area, a section-view movie showing schematically the evolution of the area is also provided. A PDF file with the animation can be downloaded from the JVE website - Download The Movie PDF.
Many features of the movie (e.g., fronts of Alps and Dinarides) are a function of plate kinematics reconstructions. Different kinematic reconstructions would imply different locations of these fronts. In the movie, Europe is kept fixed and behaves as a single plate, while Africa and Adria are moved coherently. This choice is largely under-constrained and implies that: 1) no deformation occurs within the Ionian lithosphere; 2) the rifting/drifting that drove the development of the Ionian lithosphere was completely finished at the beginning of the movie (for alternative paleogeographic reconstructions, the readers are referred to Muttoni et al., 2001; Bosellini, 2002; Conti et al., 2005); and 3) well know transcurrent features, such as the Mattinata and Tremiti faults (e.g., Tondi et al., 2005), are neglected. Also the shape and lateral continuity of the Ionian oceanic lithosphere is highly speculative.
The geometry of the link between Dinarides and Alps is perhaps the most under-constrained feature in this movie. The ancient (Paleogene-beginning of Neogene) intersection between the two mountain belts is now buried under the sediments of, or scattered within discontinuous outcrops in the Pannonian Basin. This makes it difficult to establish whether Alps and Dinarides were linked during Paleogene times. The answer is related to the choice of the paleogeography of the Mesozoic oceans that developed between Africa and Eurasia. If the oceans subducted in the Alps and Dinarides subduction zone were connected (e.g., Schmid et al., 2008), then the two belts were likely linked since the very beginning of the collisional stage. If the oceans were disconnected (e.g., Stampfli et al., 2002; Stampfli and Hochard, 2009), then no ancient connection between the two belts is necessary.
Also the location of the retreating front of the Apennines-Maghrebides and the distance between the active front and the hinge of the subducting slab are largely under-constrained. At present, geophysical and geological data suggest that this distance can vary considerably from salients to recesses of the belt. In addition, the present-day front of the various belts is highly segmented. The front was likely segmented also in the past, although the fronts are shown as linear features in the movie.
Alps
After a long period of divergence, associated with Mesozoic rifting along the Hercynian paleo-suture (Santantonio and Carminati, 2010) and drifting of the Liguro-Piedmont-Penninic Ocean (a branch of the larger Tethyan Ocean; e.g., Stampfli et al., 2002), the convergence process between the African and Eurasian plates started at the end of the Early Cretaceous or at the beginning of the Late Cretaceous, according to different reconstructions (e.g., Smith, 1971; Dewey et al., 1973; Dewey et al., 1989; Polino et al., 1990; Rosenberg et al., 2002). Along the present Alpine area, during the Cretaceous-Paleocene, the consumption of the Tethyan ocean(s) (see Polino et al., 1990, Schmid et al., 1996, Scotese, 1991, Stampfli et al., 2002, for a discussion on Cretaceous palaeogeography) took place. Subduction is also testified by HP-LT metamorphism recorded in ophiolites and former passive margin rocks in the Alps (Spalla et al., 1996), in the northern Apennines (Rossetti et al., 1999) and in Calabria (Liberi et al., 2006). Eclogitic and blue-schist facies metamorphism in the Western Alps was recognised as an evidence of a fossil subduction zone (Ernst, 1971), soon after the development of the plate tectonics theory and leading to the reinterpretation of the Alpine-Himalayan orogenic belt as the result of subduction and collision processes (Laubscher, 1969, 1970; Dewey and Bird, 1970). The eclogite facies metamorphism in the Sesia-Lanzo (Austroalpine) and Monte Rosa (Penninic) basement slices was soon recognized as a proof that large, coherent slices of continental crust were subducted (Dal Piaz et al., 1972; Marchant and Stampfli, 1997).
Interestingly, no undisputable syn-subduction (i.e., pre-collision) igneous activity is recorded along the Alpine chain. This feature (absence of syn-subduction igneous activity) is similar to what happened in the neighbouring and coeval Pyrenean Chain. The only syn-subduction volcanic activity, located in the Veneto region (NE Italy; Fig. 11a), shows “anorogenic” geochemical characteristics that are not compatible with a subduction-related origin and are rather inconsistent with the regional geodynamic setting – the foreland of the Southern Alps. Hereafter, the term anorogenic is used following the rationale proposed by Wilson and Bianchini (1999), Lustrino and Carminati (2007) and Lustrino and Wilson (2007). The term “orogenic” is similarly based on the rationale proposed by Wilson and Bianchini (1999) and Lustrino et al. (2011). What we want to stress is that geochemical terms cannot automatically be exported as geological concepts. There is no ultimate and absolutely valid geochemical or mineralogical tool to be used to define a given igneous rock as orogenic (or “subduction-related”) or anorogenic (or “intra-plate-like”). Typically, but with several exceptions, the anorogenic and orogenic igneous rocks can be distinguished considering the following geochemical characteristics: a) anorogenic rocks show lower SiO2 contents (typically <50wt.%) than orogenic rocks (typically >45 wt%); b) anorogenic rocks typically have K2O/Na2O ratios < 1.5 (commonly <1), whereas orogenic rocks have K2O/Na2O ratios from 1 to >10; c) anorogenic rocks have typically TiO2 >1.2 wt. %, while orogenic rocks have TiO2 <1.5 wt.%; d) anorogenic rocks have relatively lower LILE (Large Ion Lithophile Elements) content compared to orogenic rocks; e) anorogenic rocks have lower LILE/HFSE (High Field Strength Elements) ratios and higher HFSE contents than orogenic rocks; f) anorogenic and orogenic rocks have typically 87Sr/86Sr isotopic ratios lower and higher than BSE (Bulk Silicate Earth estimate = 0.70445), respectively; g) anorogenic and orogenic rocks have typically 143Nd/144Nd isotopic ratios higher and lower than ChUR (Chondritic Uniform Reservoir estimate = 0.51264); h) anorogenic rocks have slightly higher 208Pb/204Pb for a given 206Pb/204Pb ratio compared to orogenic rocks; i) anorogenic rocks have 207Pb/204Pb typically <15.66 (down to ~15.49) compared to values of >15.65 (up to 15.71) of orogenic rocks; j) anorogenic rocks have typically σ18O values around 5.2-5.5‰, lower than typical values of orogenic rocks (up to 8-10). For a more complete treatment of these arguments the readers are referred to Lustrino et al. (2011).
It has been claimed in the literature (e.g., Polino et al., 1990) that indirect evidence for subduction-related volcanism is preserved in the volcanic clast-rich turbidites of the Taveyanne Sandstone Formation (Savoie, France; Fig. 11b-c), originally attributed to Eocene (43 ± 4 Ma; Vaugnat, 1983). However, later geochronological 40Ar/39Ar datings on volcanic amphiboles and biostratigraphic dating on calcareous nannofossils associated with these rocks yielded ages of ~32-29 Ma (Ruffini et al., 1997 and references therein), unequivocally proving that this volcanism was syn-collisional (i.e., occurred after the end of the subduction process). Given its position and the lateral continuity with the coeval Provence volcanism, the Taveyanne volcanism could also be related to the Apenninic subduction, that will be later discussed.
Until the Eocene, the Alpine chain extended from present day Alps to the Betic Chain in SE Spain (see the movie). The continuity of the belt can be reconstructed restoring to their original position the Corsica, Calabria, Peloritani Mts. and Kabilies terranes, that display clear evidence of Alpine deformation in terms of age and tectonic transport (Michard et al., 2006; Molli, 2008; Vignaroli et al., 2009; Heymes et al., 2010, and references therein). The Europe-Adria collision took place diachronously during Eocene-Oligocene? time (Stampfli et al., 1998; Handy et al., 2010), after the complete subduction of the Ligurian-Piedmont-Penninic Ocean beneath the Adriatic continental lithosphere (Schmid et al., 1996). Strain rate decreased after the onset of collision due to decreasing convergence rates (Schmid et al., 1996). The collision brought to a phase of regional Barrowian metamorphism, characterized by high grade in the axial parts of the belt (e.g., Lepontine Dome and Tauern Window) and by decreasing grade moving toward the outer parts of the fold-and-thrust belt. As discussed, exhumation of such metamorphic rocks occurred with different patterns in Eastern (stationary exhumation) and central-western (migrating exhumation) Alps. This different behaviour has been related to north directed, lower crustal wedging in the middle and upper crust of the Central and Western Alps. The absence of such lower crustal wedge in the Eastern Alps is possibly associated to the smaller shortening and consequent smaller exhumation in the same area. In other words, the deep structure and rheology of the orogen, associated with the total shortening, and not lateral changes in its erosional efficiency, provided the main control on the lateral growth of the Alps (Rosenberg and Berger, 2009).
The continued convergence between European and Adriatic plates during post-collisional time led to accumulation of shortening and continuous enlargement of the double vergence belt (Fig. 17), with the involvement of Mesozoic and Tertiary sediments belonging to previous European and Adriatic passive margins and foredeep and foreland basin (Po Plain and Molasse basins) sediments.
In the Early Oligocene (~33-29 Ma) the Alps were characterized by a sharp increase of igneous activity with emplacement of abundant calcalkaline plutonic and subvolcanic rocks (Fig. 11b). These rocks are generated by both mantle (only Adamello gabbros, essentially) and hybrid mantle + crustal sources (the bulk of the plutons; e.g., von Blanckenburg and Davies, 1995), as evidenced by their variable 87Sr/86Sr initial isotopic ratios (~0.704-0.716) and 143Nd/144Nd initial values (~0.5128-0.5121; Fig. 18). The Periadriatic-Insubric plutons show Δ7/4 and Δ8/4 values (Fig. 19a) ranging from ~+4 to ~+80 and from ~+30 to ~+87, respectively, a feature that is commonly related to recycling of ancient sediments (Hawkesworth et al., 1986), delaminated lithospheric mantle (e.g., Mahoney et al., 1992) or lower continental crust (e.g., Escrig et al., 2004).
The Δ7/4 and Δ8/4 values represent the vertical distance of 207Pb/204Pb and 208Pb/204Pb, respectively, compared to a given 206Pb/204Pb value lying on the Northern Hemisphere Reference Line (NHRL) in207Pb/204Pb vs. 206Pb/204Pb and 208Pb/204Pb vs. 206Pb/204Pb isotopic spaces, respectively. The NHRL is considered to represent the Pb isotopic composition of oceanic basalts of the northern hemisphere of the Earth (Hart, 1984).
What is the origin of the Insubric plutons and why no subduction-related igneous activity is recorded along the Alpine Chain? The slab break-off hypothesis - that associates the magmatism with the rupture of the slab subducting under the Alps, a process that eventually led to the upwelling af mantle and to its partial melting - is the most accepted petrogenetic model to explain the igneous activity along the Periadriatic Line. Von Blanckenburg and Davies (1995) proposed this model to explain the linear distribution of the Periadriatic magmatism, the short and nearly coeval timing of emplacement of nearly all plutons, and the mantle-related source of the magmas. However, it has been argued that the linear distribution in map view does not necessarily reflect the shape of the source region, but only that of a tectonically-controlled ascent pathway (Rosenberg, 2004). The nearly horizontal rupture in the down-going slab would occur when relatively light continental lithosphere enters the trench, after continental collision. This would produce opposing buoyancy forces at depths creating strong extensional forces within the slab, ultimately tearing off and detaching the dense oceanic slab (von Blanckenburg and Davies, 1995). Based on the results of seismic tomography, Lippitsch et al.(2003) observed a spatial gap in the slab presently subducted below the Alpine orogen, interpreting this as the evidence for the slab break-off occurred during Late Eocene-Early Oligocene times. However, this interpretation is based on a 1D P-waves velocity model and can be questioned on the basis that continental subduction has been documented in the Alps down to 150-200 km (Panza et al., 1992). Moreover, the occurrence of the detachment in the tomographic models is a function of the adopted velocity model. The dehydration of the European downgoing continental segment of the slab could alternatively account for 1) the melting of the upper plate lithospheric mantle, 2) the crustal contamination of magmas, and 3) the slightly seismically slower lithospheric mantle in the upper plate, which may mimic a slab detachment (e.g., Doglioni et al., 2009).
Figure 17. Seismic cross sections through Alps and Apennines (modified after Doglioni et al., 2007).
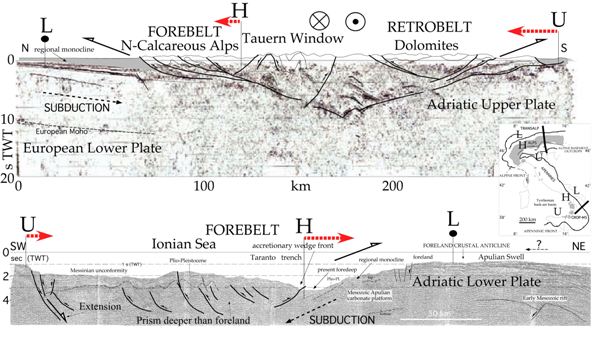
Note the Alpine double vergence (upper section), whereas the Apennines (lower section) have a single vergence. The Apennines prism is deeper than the foreland and characterized by extension at its rear (to the left). L, lower plate, H, subduction hinge, U, upper plate. Assuming fixed the lower plate (Europe for the Alps, and the Adriatic plate for the Apennines), the subduction hinge the subduction hinge is advancing towards the upper plate in the Alps and retreating in the Apennines.
Figure 18. Sr-Nd isotopic ratios of the Italian Cenozoic igneous rocks
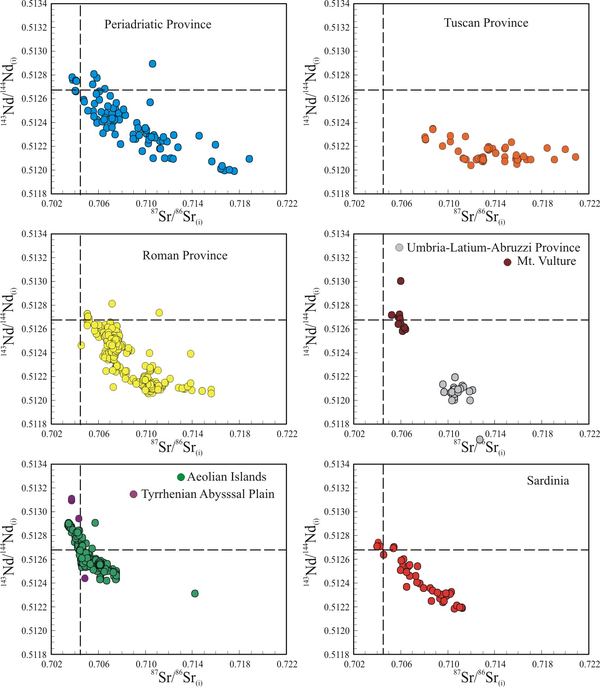
Top (a) = 143Nd/144Nd vs. 87Sr/86Sr initial isotopic ratios for the Cenozoic subduction-related (or “orogenic”) igneous rocks of Italy. BSE = Bulk Silicate Earth estimate (87Sr/86Sr = 0.70445). ChUR = Chondritic Uniform Reservoir estimate (143Nd/144Nd = 0.51264).
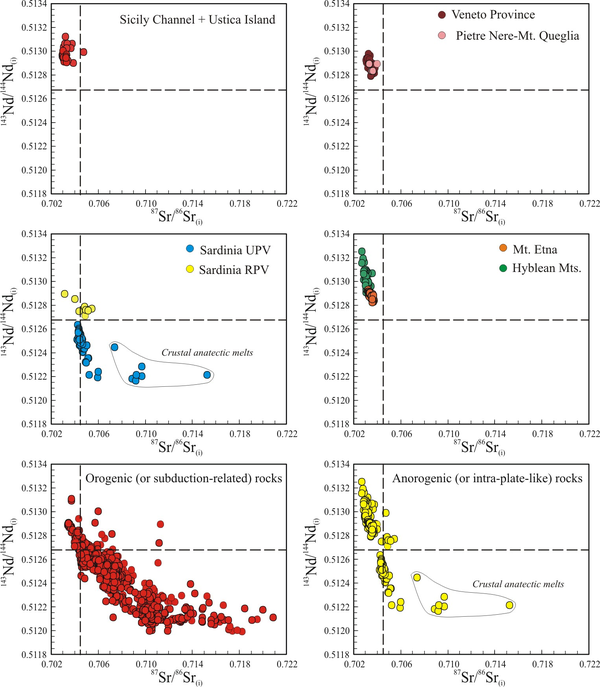
Bottom (b) = 143Nd/144Nd vs. 87Sr/86Sr initial isotopic ratios for the Cenozoic intra-plate-like (or “anorogenic”) igneous rocks of Italy. The samples enveloped in the thin continuous line are crustal anatectic rhyolites and trachytes from Mt. Arci (Sardinia) considered pure crustal (not mantle) partial melts. References for subduction-related rocks in Lustrino et al. (2011). References for intra-plate-like rocks in Lustrino and Wilson (2007) plus updates downloadable from the GEOROC web site (http://www2.mpch-mainz.mpg.de/~geo/Databases/GEOROC/Expert_Datasets.htm). The full list of analyses and references can be requested to the second author.
Figure 19. Pb-Sr-Nd isotopic ratios of the Italian Cenozoic igneous rocks
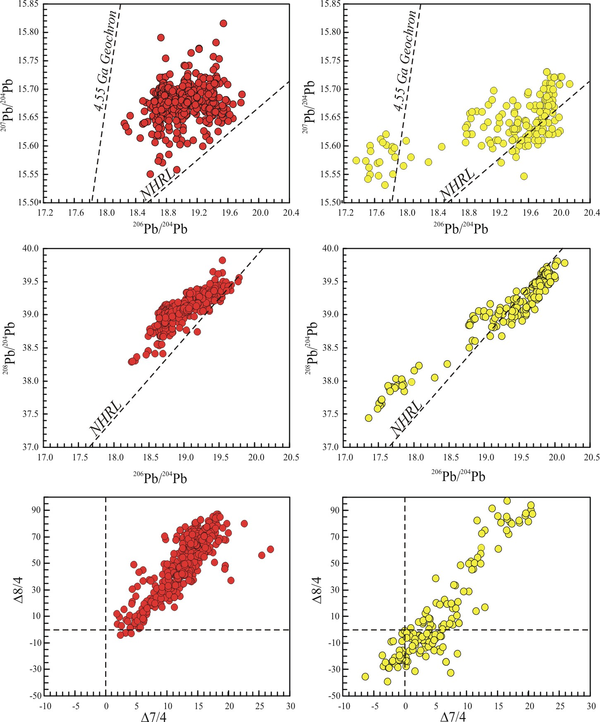
Top (a) = 207Pb/204Pb vs. 206Pb/204Pb, 208Pb/204Pb vs. 206Pb/204Pb and Δ8/4 vs. Δ7/4 diagrams for subduction-related (red circles) and intra-plate-like (yellow circles) Cenozoic igneous rocks of Italy. Δ8/4 and Δ7/4 parameters reflect the vertical shift of 208Pb/204Pb and 207Pb/204Pb from the NHRL (Northern Hemisphere Reference Line; Hart, 1984) for a given 206Pb/204Pb, respectively. The NHRL is plotted for reference in the first four diagrams. Also shown the 4.55 Ga Geochron.
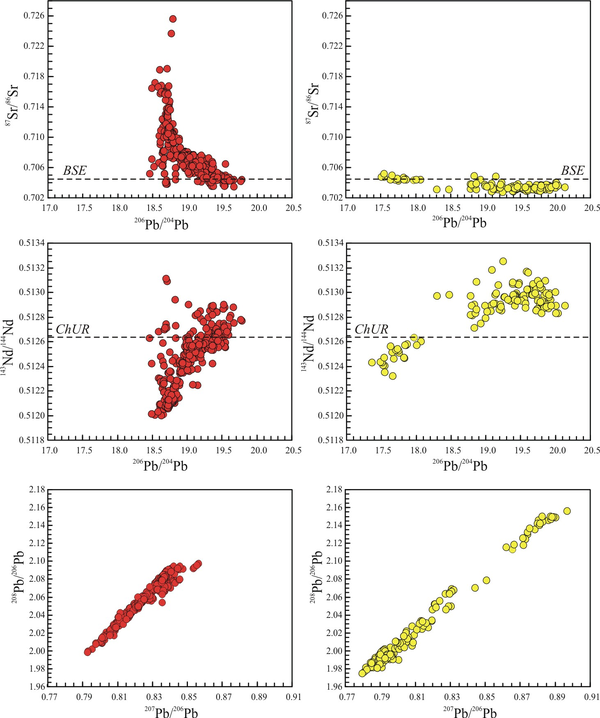
Bottom (b) = 87Sr/86Sr vs. 206Pb/204Pb, 143Nd/144Nd vs. 206Pb/204Pb and 208Pb/206Pb vs. 207Pb/206Pb diagrams for subduction-related (red circles) and intra-plate-like (yellow circles) Cenozoic igneous rocks of Italy. References for subduction-related rocks in Lustrino et al. (2011). References for intra-plate-like rocks in Lustrino and Wilson (2007) plus updates downloadable from the GEOROC web site (http://www2.mpch-mainz.mpg.de/~geo/Databases/GEOROC/Expert_Datasets.htm). The full list of analyses and references can be requested to the second author.
Figure 20. Primitive mantle-normalized multielemental diagram of the most mafic Italian Cenozoic igneous rocks
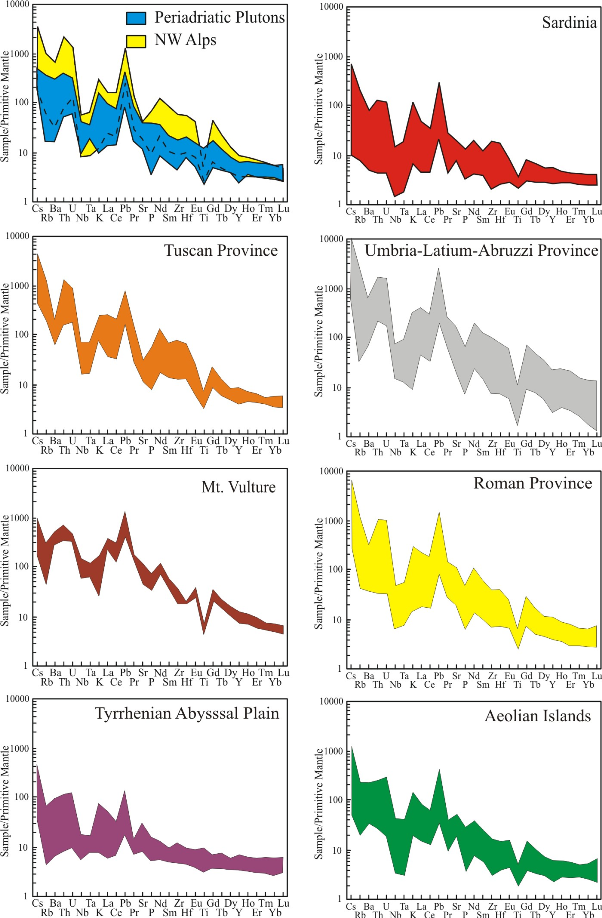
Top (a) = subduction-related (or “orogenic”) rocks. References in Lustrino et al. (2011).
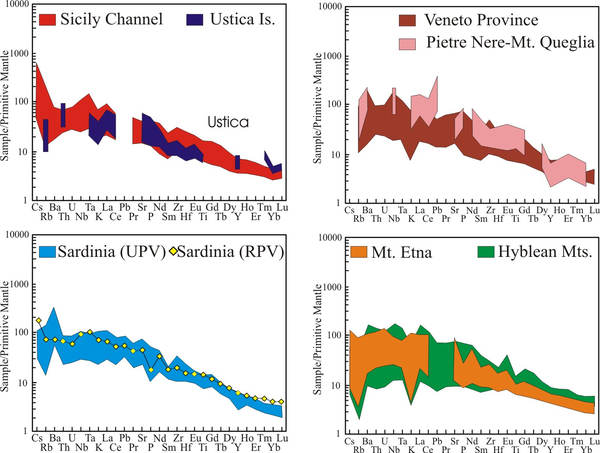
Bottom (b) = intra-plate-like (or “anorogenic) rocks. References in Lustrino and Wilson (2007) plus updates downloadable from the GEOROC web site (http://www2.mpch-mainz.mpg.de/~geo/Databases/GEOROC/Expert_Datasets.htm). The full list of analyses and references can be requested to the second author.
Less clear is the absence of subduction related igneous activity during the subduction of Tethyan oceanic lithosphere. Von Blanckenburg and Davies (1995) explained this feature as consequence of the slow pre-Oligocene convergence rates between Adria and Europe (<1 cm/yr).
The rare dykes intruding the Periadriatic plutons show commonly exotic chemical and mineralogical composition, with K content increasing from the SE Alps (mostly tholeiitic to calcalkaline compositions) to the Central Alps (mostly high-K calcalkaline) and NW Alps (mostly shoshonitic to ultrapotassic; Beccaluva et al., 1983). The NW Alps ultrapotassic dykes show a peculiar composition being mostly mafic (e.g., MgO ~6-16 wt%; high Cr and Ni content) but showing Sr-Nd isotopic composition typical of continental rocks. The paradox is that their MgO, Cr and Ni content indicate equilibrium with peridotitic assemblages, but their isotopic signal (87Sr/86Sr ~0.706-0.719; 143Nd/144Nd ~0.5120-0.5124; Fig. 19a) reflects a crustal source. All the NW Alpine dykes (high-K calcalkaline, shoshonitic and lamprophyric/lamproitic) show a typical enrichment in Rb, Th, U, Pb and LREE and depletion in Nb, Ta, Hf, Zr and Ti in primitive mantle-normalized diagrams (Fig. 20a). These geochemical characteristics allowed to infer an anomalously enriched mantle source for these magmas related to sediment input into the mantle (e.g., Venturelli et al., 1984; Peccerillo and Martinotti, 2006; Owen, 2008; Conticelli et al., 2009). Recycling of sediment (and upper crustal lithologies in general) should have happened during the eo-Alpine phase (Early Cretaceous-Middle Eocene) prior to the Europe-Adria collision.
A further complication to the evolution of the Alpine collision has been proposed for the Eastern Alps. In the Tertiary, strike-slip tectonics was abundant in this area, and was ascribed to an eastward lateral extrusion process (e.g., Ratschbacher et al., 1991). As an alternative, it is proposed that strike-slip tectonics may be explained by the interplay between the Alpine, Dinaric subductions and the transtension associated with the Pannonian rift development.
Alpine compressional tectonics recorded in the offshore east of Sardinia (Sartori, 2005) in north-eastern Corsica (Molli, 2008), Liguria (NW Italy; Vignaroli et al., 2008) and in Calabria-Peloritani Mts. (Vignaroli et al., 2009; Heymes et al., 2010) were later superimposed by the western Mediterranean rifting stage associated with the Apennines subduction back-arc. A similar tectonic scenario can be envisaged also for the Eastern Alps. The E-W extensional tectonics in this region may be linked to the back-arc extension associated with the “eastward” retreating Carpathian subduction.
Apennines, Sardinia, Tyrrhenian Sea and Sicily Channel
As shown in Fig. 2, the continental lithosphere of the Adriatic micro-plate is subducting both eastward (beneath the Dinarides) and westward (beneath the Apennines; Carminati and Doglioni, 2005). The Apennines are classically interpreted as the accretionary wedge developed along the subduction hinge of the Adriatic continental and Ionian oceanic plates. The total amount of North-South Africa/Europe convergence at the Tunisia longitude is about 135 km in the last 23 Ma, i.e., about five times smaller than the “eastward” migration of the Apenninic subduction hinge, which moved eastward more than 700 km during the same interval (Gueguen et al., 2008). Therefore the slab retreat cannot be ascribed to the Africa-Europe convergence. It must rather be controlled by the slab-mantle intercation, i.e., the slab pull or, alternatively, to the eastward mantle flow relative to the lithosphere. This “eastward” rollback of the Apenninic subduction produced the back-arc stretching of the lithosphere of the Ligurian-Provençal Basin and the Tyrrhenian Sea, with oceanization in the Vavilov and Marsili sub-basins (e.g., Malinverno and Ryan, 1986; Doglioni 1991).
The Apennines subduction zone (with a slab dipping even steeper than 70° along the Calabrian arc; Giardini and Velonà, 1991; Carminati et al., 2002, 2005; Chiarabba et al., 2008) is considered connected, through Sicily and the Sicily Channel, to the North Africa Maghrebides (Rif and Tell Orogens) subduction. Geophysical and igneous petrological considerations provide evidence of the subduction of the Adriatic and Ionian lithospheres underneath the whole Apennines (e.g., Peccerillo, 1985, 2005; Royden et al., 1987; Selvaggi and Amato, 1992; Serri et al., 1993; Selvaggi and Chiarabba, 1995; Piromallo and Morelli, 1997; De Gori et al., 2001; Avanzinelli et al., 2009; Lustrino et al., 2011). Seismic data, moreover, permit to recognize the continental subduction in the north, from the oceanic subduction in the south (e.g., Catalano et al., 2001). The slab has been shown to be continuos and undetached under Calabria (Mele, 1998).
Based on the aforementioned structural and petrologic pieces of evidence, the Paleozoic highly metamorphosed Alpine-age terranes in Calabria and Peloritani Mts. are considered as a part of the Alpine belt that was dismembered during the opening of the Ligurian-Provençal and Tyrrhenian Sea basins from Early Oligocene onwards. The paleo-geographic location of the Calabrian-Peloritani Mts. continental crust is still a matter of debate. It has been proposed to be part of: 1) the European southern paleo-continental margin (Ogniben 1969; Knott, 1987; Dietrich, 1988); 2) the African paleo-margin (Alvarez, 1976; Amodio Morelli et al., 1976); 3) an intervening micro-plate (Doglioni, 1991; Guerrera et al., 1993, 2005; Cello et al., 1996). The present-day Apennines-related deformation front of the accretionary prism is located offshore in the Ionian basin (Doglioni et al., 1999).
In summary, the Apennines Chain is interpreted as being related to a subduction process involving recycling of continental and oceanic lithosphere and thrusting of different types of lithologies (Meso-Cenozoic carbonatic and deep oceanic basin successions). Evidence for active subduction beneath the Apennines is suggested by local tomographic studies (De Gori et al. 2001) and the absence of sub-crustal seismicity within the continental lithosphere of the Adriatic plate subducting under the southern Apennines could be rheologically controlled (Carminati et al., 2002, 2005).
The “westward” subduction and “eastward” retreat of the Ionian oceanic lithosphere and the Adriatic continental lithosphere started probably during Early-Middle Eocene and is largely still active (Devoti et al., 2008). The occurrence of ~38 Ma-old subduction-related volcanic rocks in Sardinia led Lustrino et al. (2009) to propose a beginning of subduction between 42 and 49 Ma ago. The age difference between the first magmatic activity and the beginning of the subduction system depends on the relative plate motion, the dipping angle of the subducting slab and the depth at which partial melt occurs in the mantle wedge. The 42-49 Ma age for the beginning of the Apennine subduction system is far older than the 30 Ma age previously proposed (Réhault et al., 1984; Malinverno and Ryan, 1986; Ricci Lucchi, 1986; Patacca and Scandone, 1989; Boccaletti et al., 1990; Gueguen et al., 1998; Carminati et al., 1998). The 30 Ma age was mainly based on previous geochronological estimates available for subduction-related igneous rocks and on the age of syn-tectonic sediments in the western Mediterranean basins. The discrepancy between the age of extensional tectonics and magmatism suggests that the mechanical response to the retreat of the subducting slab is more sluggish than the thermal-petrological response. In addition, such a discrepancy can be also related to the fact that extensional tectonics in the western Mediterranean back-arc basins generally impacted a pre-existing over-thickened lithosphere (Alpine-Betic subduction), apart west of Sardinia, where the rift enucleated in the foreland of the Alps. Thus the first extensional motions, that disrupted the previous topography, were likely associated with the development of irregular continental basins. As a consequence, the sedimentation associated with these early stages may have been discontinuous and the precise dating of such sediments would not be possible.
During Eocene-Oligocene times a critical scenario developed in the proto-western Mediterranean area, with the existence of the S-SE-directed Alpine subduction system (approaching the end of its existence) and the young NW-directed Apennine subduction system (e.g., Carminati et al., 1998; Doglioni et al., 1998; Vignaroli et al., 2008; Lustrino et al., 2009), which possibly developed along the retrobelt of the Alpine orogen (Fig. 21). This means that two subduction systems, with nearly opposite polarity, were present in a relatively narrow area for a short time (Fig. 22).
The southern prolongation of the Western Alps has been incorporated into the internal Apennines, and the Apennines slab retreat induced subsidence and boudinage of large portions of the Alps, partly counteracting their uplift (Fig. 22). From the Marittime Alps southwards, the Alps have been scattered and dismembered into the Apennines-related backarc. However, at least until the Middle Miocene, the Alps continued to be active, in spite of being stretched by the back-arc rift of the ongoing Apennines subduction. This seems testified by the lower Miocene HP/LT rocks of the Tuscan area, an assemblage that can form only where the crust is thickened as in the Alpine system. The coexistence of opposite subduction zones is documented also in other areas, like central America and Molucche.
Figure 21. The W-directed Apenninic subduction started in the western Mediterranean in the Eocene(?)-Early Miocene along the retrobel belt of the Alps-Betics orogen (in green).
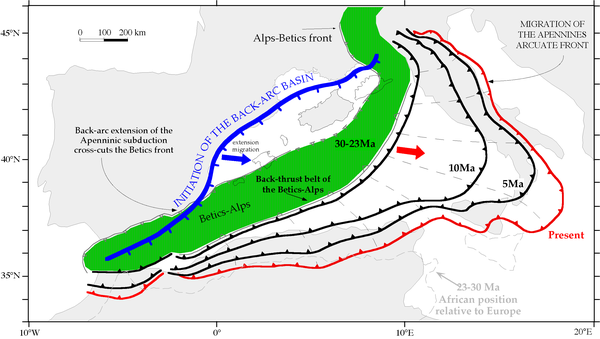
The Apennines arc migrated eastward up to the present position in association with the slab retreat. The western Mediterranean back-arc basins developed in the hangingwall of this retreating slab. Note the lengthening of the arc which should generate also along arc extension. The western border of the back-arc rifting has a convex shape, mirroring the opposite Apennines arc. The initial rift cross-cuts the Alpine-Betic front, suggesting that the extension is not simply the collapse of the Alps-Betics orogen (after Doglioni et al., 1999).
The rifting-drifting of Sardinia, and the formation of the Ligurian-Provençal Basin, as well as the emplacement of large amounts of igneous rocks have been related to the existence of the NW-directed Apennines subduction system that evolved to form the present-day Apennines belt (Carminati and Doglioni, 2005, and references therein). The geochemical composition of the Late Eocene-Middle Miocene igneous rocks of Sardinia resemble closely the magma presently generated along subduction settings, with abundant calcalkaline and high-K calcalkaline, with minor arc-tholeiitic compositions (Brotzu, 1997; Morra et al., 1997; Mattioli et al., 2000; Franciosi et al., 2003; Lustrino et al., 2004, 2009; Fig. 10b). Subduction-related coeval igneous rocks with a similar chemical composition were generated also along the Provençal coast (SE France), in SE-Corsica and offshore SW-Corsica. The volume and the areal extent of these products are about two orders of magnitude smaller than the volume of the Sardinian rocks. These features are probably related to the minor stretching rate in Provence and Corsica, located closer to the rotation hinge near the Gulf of Genoa (Fig. 1). During the 35-30 Ma interval, a nearly continuous NE-SW-trending volcanic line developed in the Western Mediterranean, with emplacement of subduction-related volcanic rocks in the Malaga area (S Spain; Duggen et al., 2004), Mallorca island (Balearic Islands; Martì et al., 1992), Sardinia (Lustrino et al., 2009) and Provence (SE France; Beccaluva et al., 2004). These rocks can be considered as the trace of the Apennines paleo-trench system (Lustrino et al., 2011).
Figure 22. Within the Cenozoic evolution of the Mediterranean, Italy was shaped by the Alpine and Apeninnes subduction zones.
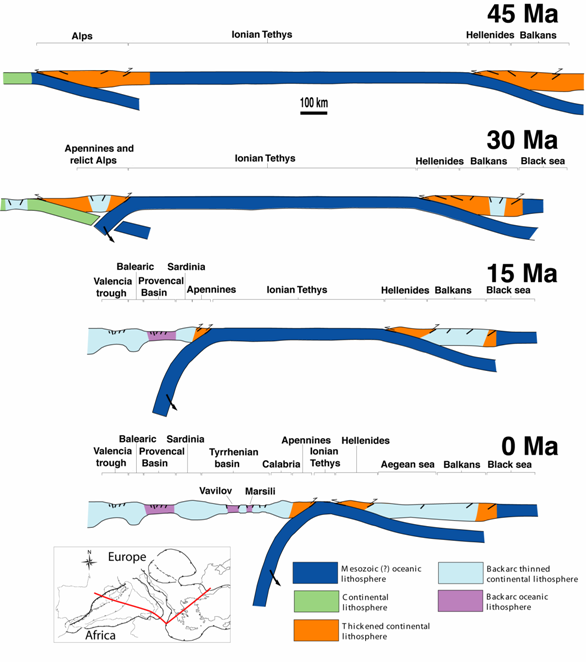
The early “east”-directed Alpine subduction was followed by the Apennines “west”-directed subduction,which developed along the retrobelt of the pre-existing Alps. The slab is steeper underneath the Apennines, possibly due to the “westward” drift of the lithosphere relative to the mantle (after Carminati and Doglioni, 2005).
During Late Eocene-Middle Miocene times, the arc magmatism was almost exclusively confined to Sardinia, in the upper plate of the Apennines subduction. Other minor areas interested by subduction-related igneous activity are the Early Oligocene Po Plain in the Apennine foreland (Mortara buried volcano; ~30 Ma; Mattioli et al., 2002a; Fig. 11c) and the nearly coeval (~29 Ma) conglomerates rich in volcanic fraction of northern Apennines (Aveto-Petrignacola Formation; Mattioli et al., 2002b; Fig. 11c). The subduction-related igneous activity continued until Middle Miocene (~15 Ma; Fig. 11b-c) only in Sardinia. During Middle to Late Miocene (~15-7 Ma), no important igneous activity developed in the Italian area, whereas the westernmost Mediterranean was characterized by abundant subduction-related igneous activity in the Betics and Rif Belts (Turner et al., 1999; Duggen et al., 2004, 2005; Doblas et al., 2007).
As outlined previously, starting from the latest Miocene - but essentially during Plio-Quaternary times - another phase of “subduction-related” igneous activity developed along peninsular Italy, from Tuscany (northern Apennines) to Campania (southern Apennines) regions, along the Tyrrhenian Sea border. This activity, almost entirely characterized by products with potassic to ultrapotassic composition, possibly witnesses the continental nature of the slab sinking beneath the Apennines. The origin of the potassic and ultrapotassic rocks of the Roman district (the so-called Roman Comagmatic Province of Washington, 1906) has been classically ascribed to partial melting of an heterogeneous mantle sources with phlogopite-rich veins originated after interaction of slab-derived melts and fluids with ambient mantle (Peccerillo, 1985, 1999, 2005; Beccaluva et al., 1991; Conticelli et al., 2002, 2004, 2009; Avanzinelli et al., 2009, and references therein). In particular, the leucite-bearing magmas have been modelled with a process of interaction of sediments (essentially marls) brought at mantle depths during subduction processes (e.g., Peccerillo, 1985; Beccaluva et al., 1991; Avanzinelli et al., 2009, and references therein). In this case the mantle source should be lherzolitic to wehrlitic, in order to explain the CaO-rich composition of kamafugites and leucite-bearing ultrapotassic rocks. Experimental petrology studies evidence that melting of this type of lithologies under high XCO2 can produce liquids resembling kamafugites and other SiO2-undersaturated ultrapotassic rocks even at relatively low pressures (see discussion in Avanzinelli et al., 2009). The enrichment in Ca and CO2, as well as the low SiO2 content of the magmas, and the abundant CO2 emission along the Italian peninsula and near the Calabria-Peloritani arc are considered to reflect interaction with CaCO3-rich recycled sediments in a mantle wedge (e.g., Avanzinelli et al., 2009; Frezzotti et al., 2009, and references therein).
During the eastward retreat of the Apennines slab the upper plate, including the inherited Alpine orogen, has been sheared and boudinaged (Fig. 23). The retreat of the slab implies that asthenospheric mantle had to compensate the volume left by the migrating subduction. In other words, an eastward mantle flow has to be envisaged in the Western Mediterranean basin since the onset of the Apennines subduction, irrespective of whether it the cause of the slab retreat or the consequence. The shear-wave splitting analysis of this area (Lucente et al., 2006) confirms a relevant seismic anisotropy that can be associated with mantle flowing from west to east in the Mediterranean and encroaching a slab, which constitute an obstacle deviating the flux (Fig. 24).
The boudinage and rifting in the Tyrrhenian Sea in the hangingwall of the subduction generated subsidence in the backarc, uplift above the subduction zone, and subsidence in the foredeep (Fig. 15). A number of different mechanisms controlled contemporaneously the vertical movements in the area. Lateral migration of depleted mantle can determine uplift; stretching and thermal cooling in the basin rather generates subsidence; mantle wedging and accretion above the subduction hinge produce uplift; slab rollback generates subsidence in the foredeep; loading of the prism and the foredeep trigger subsidence, etc. (Fig. 16). Therefore different geodynamic mechanisms may coexist along a cross-section showing that not a unique mechanism is determining the vertical movements, and that the subduction system may rather be considered as a passive process.
In this scenario, the down-flexure induced by the Apennines subduction (Fig. 15) explains the fast subsidence rates in the Po Plain and in the northern and central Adriatic Basin (Carminati et al., 2003a).
The Pleistocene-Present uplift of the southern Adriatic Basin and of Apulia is explained by the entrance in the subduction zone of 110 km-thick continental lithosphere that chocked the subduction and induced buckling of the subducting lithosphere (Doglioni et al., 1994). The fact that the highest mountains do not coincide with the water divide is interpreted as induced by the faster ‘eastward’ propagating tectonic wave generated by the retreat of the Apennines subduction zone during Pliocene and Quaternary times (10-30 mm/a) with respect to average denudation rates (<1 mm/a) (Figs. 12 and 13).
Figure 23. The western Mediterranean shows large asymmetric scale boudinage of the lithosphere in the back-arc extensional setting which developed particularly in the last 30-40 Ma.
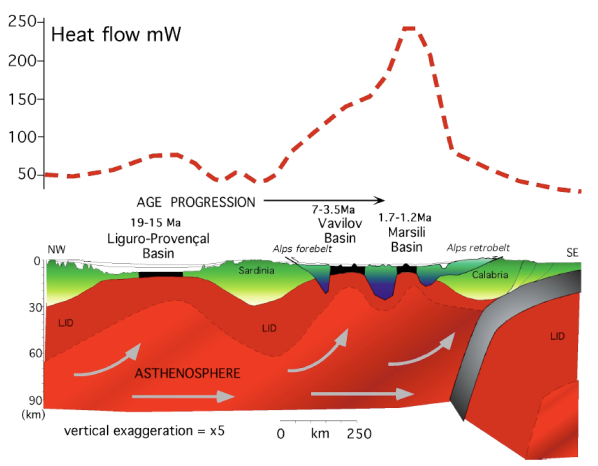
The age progression from west to east is indicate by the eastward younging of the basins. The most active spredingis occurs the Tyrrhenian Sea where there is also the highest heat flow. The backarc extension in the Tyrrhenian Sea stretched the pre-existing Alpine double-verging orogen (dark blue and green). The “eastward” retreat of the Apennines slab has been compensated by the eastward mantle flow in the hangingwall. The oceanic crust is shown in black (after Gueguen et al., 1997; Zito et al., 2003).
Models involving nearly horizontal ruptures of a slab (slab break-off; e.g., De Astis et al., 2006; Bianchini et al., 2008), vertical ruptures of a slab (slab window; e.g., Schiattarella et al., 2005; D’Orazio et al., 2007) or more complex scenarios (horizontal detachment of subducted slab in post-collisional conditions coupled with vertically-propagating tears in the slab and differential slabs roll-back; Rosenbaum et al., 2008) have been alternatively proposed to explain the climax of the igneous activity in the Mt. Vulture area and in the Roman Province. In all cases, a toroidal mantle flow would have put in contact uncontaminated African (Adriatic) foreland mantle sources with subduction-related fluids and melts related to the Apennine subduction system, generating an hybrid mantle source with subduction-related geochemical features but also with still partially visible pre-metasomatic compositions, ranging from OIB- (Ocean Island Basalts) to DMM- (Depleted MORB Mantle) like; e.g., Peccerillo, 2005; D’Orazio et al., 2007; Bianchini et al., 2008; Rosenbaum et al., 2008; Avanzinelli et al., 2009).
Figure 24. Seismic anisotropy in the central-western Mediterranean and P-wave mantle tomography at the depth of 150 km (after Lucente et al., 2006).
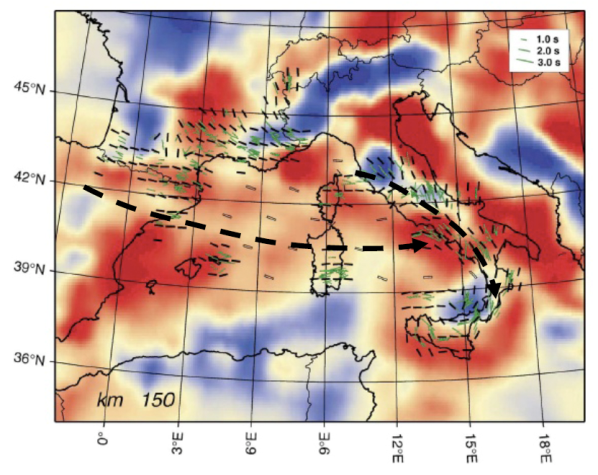
Note the alignment of the anisotropy (SKS splitting measurements) with the supposed mantle flow shown with the thick dashed black line in the hangingwall of the W-directed, easterly retreating Apennines slab. The inferred flow tends to parallel the slab when approaching the Apennines subduction zone, suggesting the presence of an obstacle able to deviate the mantle flow.
The tectonic setting of the Aeolian Islands is particular, being spatially and temporally associated to volumetrically important igneous activity in the neighbouring Mt. Etna, with emplacement of lavas with anorogenic geochemical characteristics (see discussion and references in Lustrino and Wilson, 2007). The peculiarity of this sector is based on the fact that the external area of the Aeolian Islands igneous activity (i.e., toward the trench) is occupied by the exotic terrane of Calabria (the tip of the Italian peninsula) and Peloritani Mts. (the NE-most margin of Sicily) which are separated from the Apennine-Maghrebian belt by the Sangineto and Taormina tectonic lineaments, respectively (e.g., Elter et al., 2004, and references therein). The origin of the igneous activity in the Aeolian district is considered to be related to the metamorphic reactions of the Ionian subducting plate acting at depths ranging from ~100 to 250 km in depth. These slab dehydration processes metasomatize and soften the mantle wedge, lowering its solidus temperature, favouring partial melting processes (e.g., Peccerillo, 2005, Francalanci et al., 2007; Chiarabba et al., 2008; Peccerillo et al., 2008, and references therein).
The origin of the volcanic activity of Mt. Etna shows many similarities with the Aeolian Islands, although the Aeolian volcanism is above the projection of the slab at depth (100-120 km), whereas the Etna volcano is located on the subduction hinge (Doglioni et al., 2001). This feature is at the same time obvious and unexpected. It is obvious because Mt. Etna is located only few km south of the southernmost Aeolian island and because Mt. Etna and Aeolian Islands are nearly coeval. It is surprising because the products of the two volcanic areas have completely different geochemical and isotopic characteristics, incompatible with a similar mantle source. In particular, the formation of the Mt. Etna volcano has been considered to be related to an asthenospheric flow through a slab window created as consequence of differential roll-back of the subducted Ionian (Mesogean) oceanic lithosphere relative to the neighbouring Sicilian continental lithosphere. The Malta escarpment represents a Mesozoic-Tertiary passive continental margin separating the Sicilian and Ionian domains. This area acted as a transfer zone during the slab retreat of the Apennines, allowing larger roll-back of the Ionian oceanic lithosphere with respect to the Sicilian continental slab. The differential roll-back is evident when comparing the shallow dip of foreland regional monocline in the Hyblean Plateau with the steeper dip beneath the Ionian accretionary prism (Doglioni et al., 2001). The tear separating the two different rates of rollback is concentrated along the inherited Malta escarpment, which was used during the Apennines subduction as a right-lateral transtension trasfer. This process allowed passive upwelling of asthenospheric mantle through such a vertical slab-window that generated partial melting as consequence of adiabatic decompression (e.g., Gvirtzman and Nur, 1999; Doglioni et al., 2001; Armienti et al., 2004). Such asthenospheric “suction” might also have mobilised subduction-related fluids coming from the neighbouring Ionian oceanic plate (e.g., Tonarini et al., 2001).
The Hyblean Mts. igneous rocks show many geochemical, mineralogical and isotopic similarities with the Mt. Etna products (Figs. 10, 19 and 20), but their origin must rely on different geological processes, being the tectonic settings very different. Indeed, the recent igneous activity of Hyblean Mts. has been related to extensional tectonics and the development of pull-apart basins, with subsequent adiabatic decompression melting (Trua et al., 1997; Beccaluva et al., 1998). On the basis of major and trace element and Sr-Nd-Pb isotopic constraints, Beccaluva et al. (1998) proposed that the Miocene-Pliocene volcanism of the Hyblean Plateau was caused by partial melting of a depleted mantle source, variably modified by asthenosphere-derived melts or fluids, with both alkali-silicate and carbonatitic compositions, capable of stabilizing metasomatic phases (e.g., amphibole, phlogopite, apatite and carbonate), which partly replaced the original mantle paragenesis.
As the Tyrrhenian Sea igneous rocks are concerned, it must be noted that their major and trace element composition is certainly anomalous with respect to classical oceanic floor produced in divergent settings such as North Atlantic Ocean. This testifies to the occasional presence of metasomatic modifications of their sources related to slab-derived percolating melts/fluids. The average depth of the Tyrrhenian sea-floor is comparable with sea-floor depths of Atlantic Ocean of the same age (Kastens et al., 1988; Korenaga and Korenaga, 2008).
Major and trace element content of Sicily Channel volcanic rocks is compatible with emplacement in a foreland rifted area from mantle sources that excaped subduction-related metasomatic modifications. More in detail, variable trace element ratios and elevated 207Pb/204Pb in Pantelleria rocks are believed to be indicators of the involvement of ancient enriched mantle source components (which resemble the enriched lithosphere sampled by circum-Tyrrhenian potassic magmas) in addition to DMM-HIMU (HIMU = High μ; μ = 238U/204Pb) (± EMI = Enriched Mantle type I) mantle sources (Esperança and Crisci, 1995; Civetta et al., 1998). With the exception of one sample, the total range of 87Sr/86Sr and 143Nd/144Nd is 0.7030-0.7033 and 0.51300-0.51292, respectively (Fig. 17b). Compared to the limited range of Sr-Nd isotope compositions, the Pb isotopic ratios show a much wider range (206Pb/204Pb = 18.30-19.94, 208Pb/204Pb = 38.04-39.62: Δ7/4 = -6.4 to +11.6; Δ 8/4 = -39.4 to +45.8; Fig. 19b). Trace element abundances of Linosa Island volcanic rocks (Rossi et al., 1996; Bindi et al., 2002; Peccerillo, 2005) show the typical bell-shaped patterns in primitive mantle-normalized multi-elemental diagrams, peaking at Nb with relatively low LILE abundances, within the HIMU-OIB compositional field (Fig. 20b). Sr-Nd-Pb isotopic ratios range as follows: 87Sr/86Sr from 0.7030 to 0.7031, 143Nd/144Nd from 0.51298 to 0.51295, 206Pb/204Pb from 19.28 to 19.43, 207Pb/204Pb from 15.60 to 15.62, 208Pb/204Pb from 38.89 to 39.02, Δ7/4 from +1.5 to +2.8, Δ8/4 from -12.5 to -4.3 (Figs. 17b and 19b). Esperança and Crisci (1995) proposed a petrogenetic model in which a lithospheric mantle source, enriched and metasomatized by asthenospheric partial melts, undergoes partial melting in an extensional tectonic regime without any mantle plume involvement. Corti et al. (2006) propose an origin for Pantelleria and Linosa islands related to the oblique crustal extension in the foreland of the Apennines-Magrebide front, testifying the existence of two completely different and unrelated geodynamic processes (continental extension and active subduction). This coexistence is very relevant in supporting the idea of the passive nature of plate boundaries (i.e., both their geometry and evolution are governed by forces associated to far processes), casting serious doubts on their relevance in driving plate tectonics. Volcanic rocks from Graham and Nameless Banks in the Sicily Channel have geochemical characteristics similar to Pantelleria and Linosa Islands, broadly resembling HIMU-OIB. Small differences are related to sea-water alteration (Rotolo et al., 2006). As a whole, the Sicily Channel seamounts have 87Sr/86Sr ranging from 0.70308 to 0.70478, 143Nd/144Nd from 0.51312 to 0.51295 (Fig. 17b), 206Pb/204Pb from 19.15 to 19.81, 207Pb/204Pb from 15.66 to 15.70, 208Pb/204Pb from 38.95 to 39.62, Δ7/4 from +3.9 to +13.0, Δ8/4 from -13.0 to +19.4 (Fig. 19b).
Ustica lavas have a small range in 87Sr/86Sr (0.7030-0.7033) and 143Nd/144Nd (0.51300-0.51290; Fig. 17b) and a slightly wider range of compositions for 206Pb/204Pb (18.84-19.56), 208Pb/204Pb (38.61-39.19), Δ7/4 (+3.3 to +7.4) and Δ8/4 (-7.8 to +20.8); Trua et al., 2003; Peccerillo, 2005; Fig. 19b). On the basis of their trace element and Sr-Nd isotopic compositions, Cinque et al. (1988) and Trua et al. (2003) proposed that the source of the Ustica alkali basalts was slightly contaminated by a component of slab origin. It has been suggested that the volcanic activity of Ustica (together with that of the Prometeo seamount and Mt. Etna) can be explained without the need to invoke a deep-rooted mantle plume, but considering instead the flow of African mantle below Sicily, channelled along the SW edge of the Ionian oceanic plate which is undergoing subduction roll-back (e.g., Gvirtzman and Nur, 1999; Doglioni et al., 2001; Trua et al., 2003).