4. Geochemistry
In this section we present whole-rock and mineral chemical data from samples collected across the pluton margin. Additionally, we estimate the degree to which metasomatic alteration and volumetric strain accompanied deformation, and present the generalized metamorphic reactions inferred from the observed mineralogical and microstructural changes.
4.1. Whole-rock chemistry
The bulk chemical composition of samples from the marginal Cerro de Costilla tonalite was analysed by ICP-MS at Activation Laboratories, Ontario, Canada to evaluate possible chemical variability associated with the deformation gradient (Table 1). Samples were collected at progressively smaller intervals from the undeformed interior (samples H-J), through progressively more deformed rocks (samples D-F), into the highly-sheared margin (samples A-C).
Figure . Table 1.
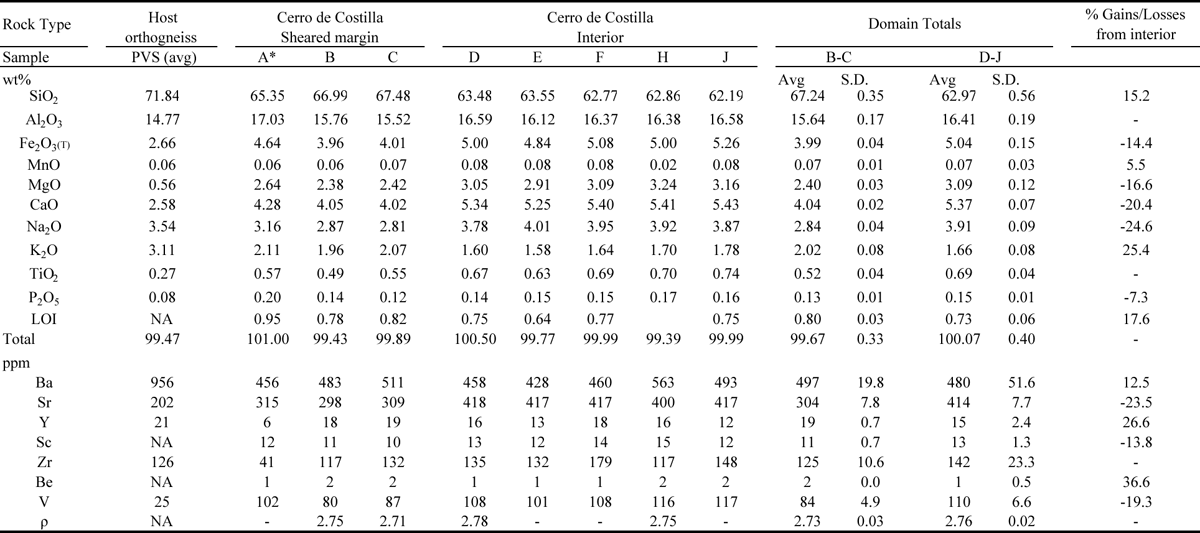
Whole-rock geochemistry and calculated bulk compositional changes in the sheared margin of the tonalite (samples B & C) based on Isocon method. PVS = published data for Pine Valley Suite orthogneisses (Todd et al., 2003). Densities calculated from sample mode and measured mineral compositions.
Samples from the less deformed rocks (D-J) have a relatively uniform, tonalitic bulk composition. Within ~1 m of the contact with the wall-rock granitic orthogneiss, a sharp change occurs in most major and trace element concentrations (Fig. 33). Samples B and C have much lower concentrations of Na2O, MgO, CaO, Fe2O3, and Al2O3, and higher SiO2, K2O, and LOI (taken as H2O) than samples D – J (Fig. 33). Sample A, collected within 10 cm of the host rock contact, has markedly more Na2O, MgO, CaO, Fe2O3, and Al2O3, and less SiO2, Y, Zr, and V, relative to adjacent samples B and C. The presence of cm-scale quartz and tourmaline-rich veins near the host rock contact suggests that this 10-20 cm wide zone may have been subject to additional fluxing of fluids, possibly with different compositions, compared with the more interior marginal samples (B and C). Sample A is therefore excluded from mass balance calculations presented below.
Figure 33. Bulk chemistry from interior to margin of the shear zone.
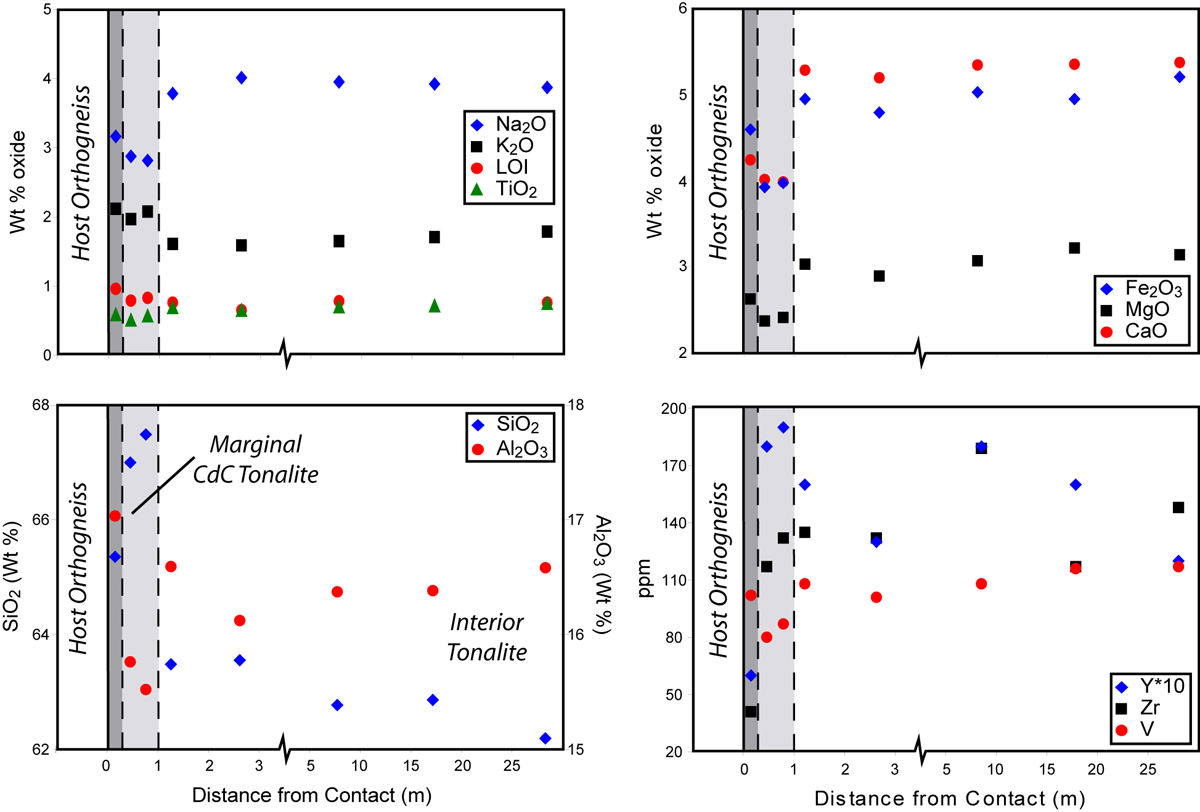
Spatial variation in bulk chemistry from interior to margin of the shear zone. Note the substantial changes in chemistry within c. 1 m of the contact with the host orthogneiss.
4.1.1. Mass-balance and volumetric strain
The relative changes in whole-rock major and trace element concentrations between the unaltered protolith (samples D-J) and the sheared marginal rocks (B and C) are evaluated using the Isocon method (Grant, 1986, 2005). The choice of a reference isocon is often problematic, and can be based on some combination of petrographic observations, statistical methods, and experimental determination of elemental behavior (see Baumgartner and Olsen, 1995 and Grant, 2005 for reviews). Low solubility and/or high field strength elements (e.g., Al, Ti, and Zr) are commonly assumed to be relatively immobile in metasomatic environments (e.g., Ague, 1994, Lonka et al., 1998, Barnes et al., 2004; Grant, 2005), although several examples of mobility of these elements have been described (e.g., Lafrance & Vernon, 1993, 1999; McLelland et al., 2000a, 2000b; Barnes et al., 2004). For this study, we select a best-fit isocon through Al2O3, TiO2, and Zr that has an excellent correlation (R2 = 0.988) and is consistent with suspected major element gains (SiO2, K2O, and H2O) and losses (Na2O, MgO, CaO, Fe2O3) based on petrographic observations and chemical data (Fig. 34). The relative enrichment and depletion of major elements calculated from the immobile element isocon are presented in Table 1, and show a ~15%-25% change in each of the mobile major oxides.
Figure 34. Isocon plot of average interior and marginal bulk compositions across the shear zone.
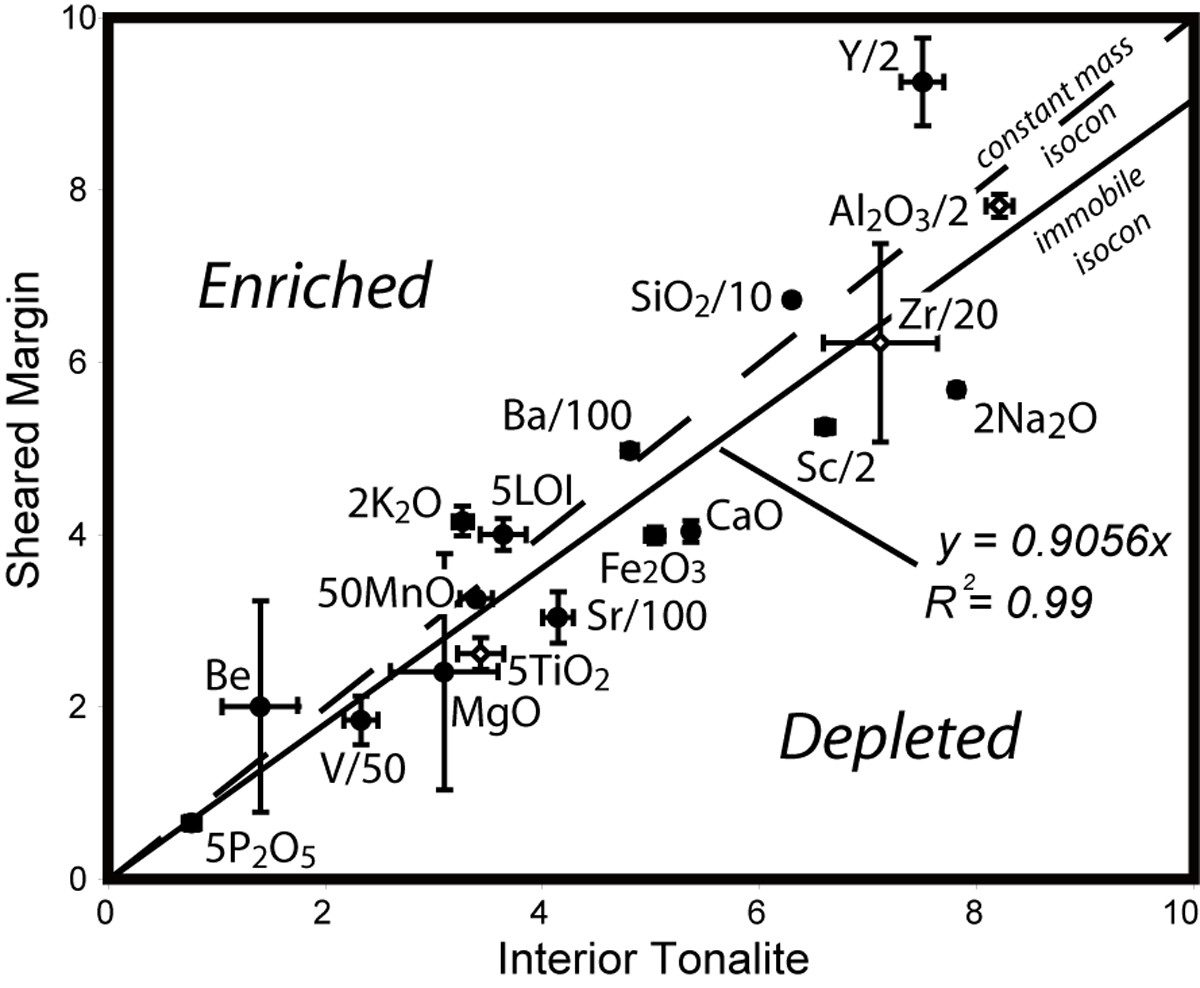
Isocon plot of average interior and marginal bulk compositions across the shear zone. Note the enrichment in K2O, SiO2, and LOI, and depletion in Fe2O3, CaO, and Na2O within the sheared margin relative to the immobile isocon.
The slope of the isocon (0.9056) indicates an overall mass gain of ~10% during metasomatic alteration of the tonalitic protolith (Grant, 1986). The equation:
(Grant, 1986) yields an approximate volumetric strain of +12 %, owing to the minor decrease in the average densities (Table 1 ) of altered samples (B and C), relative to protolith samples (D and H), as calculated from modes (Table 2). Thus, a dilational component of deformation in the pluton margin is inferred from the mass-balance evidence.
4.2. Mineral chemistry and inferred reactions
In addition to the microstructural and bulk chemical changes across the sheared pluton margin, systematic changes in plagioclase, biotite, and hornblende composition are also observed (Fig. 35, Table 3). The major modal changes from the undeformed interior to the most deformed rocks are shown in Fig. 36 and include: a steady decrease in plagioclase, a sharp decrease in hornblende, and substantial increases in biotite and quartz in marginal samples (Table 2). Equations intended to represent localized mass transfer or reactions inferred from petrographic observations and associated compositional changes in plagioclase, biotite, and hornblende are discussed below.
Figure 36. Modal mineralogy from the interior to the margin of the shear zone.
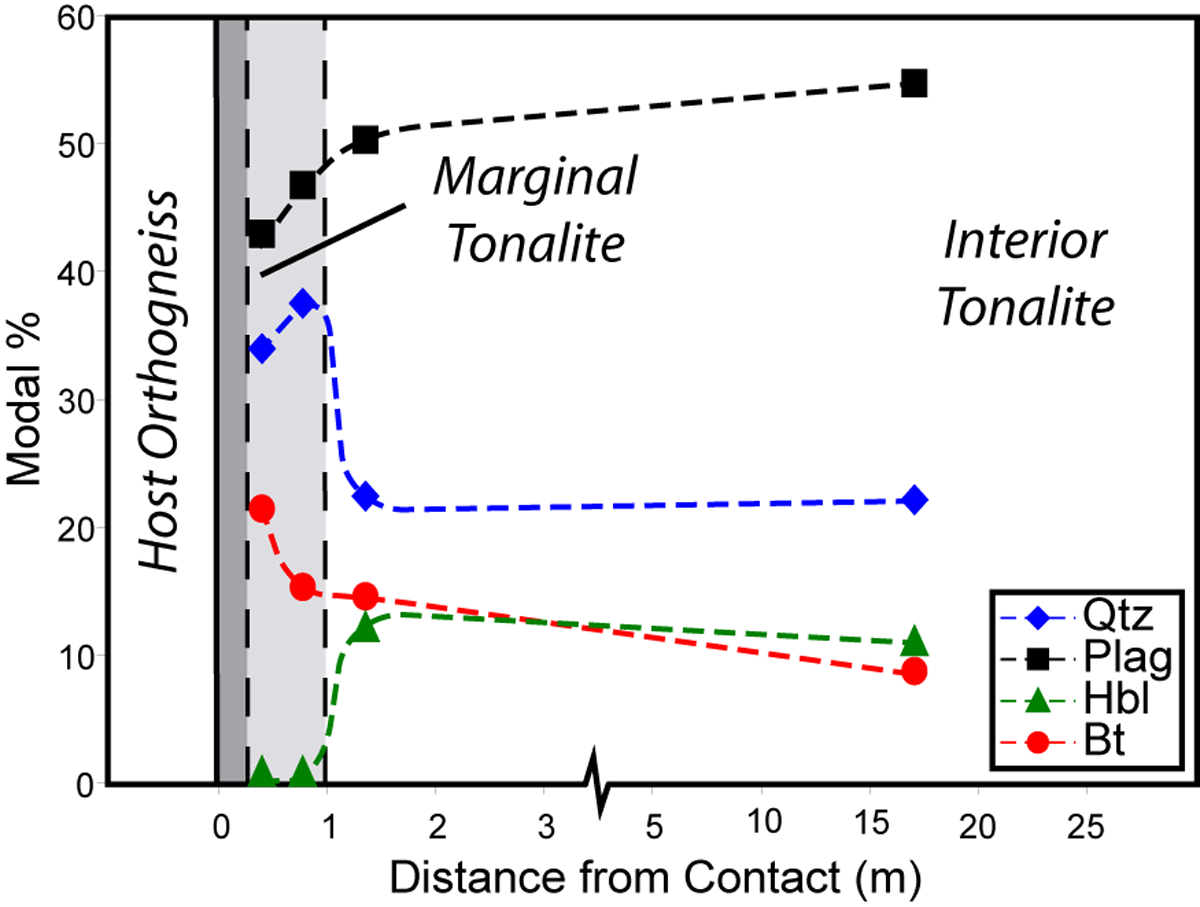
Spatial variation in modal mineralogy from the interior to the margin of the shear zone. Note the marked changes in modal mineralogy within ~ 1m of the contact with the host orthogneiss.
4.2.1. Plagioclase
In the least deformed tonalite, coarse-grained plagioclase preserves its original magmatic compositional zoning, with XCa fluctuating by up to 0.10 within single grains, and an average of 0.37. Small plagioclase grains are typically unzoned and consistently more sodic than the larger zoned grains, with an average XCa of 0.30 (Fig. 35).
Close to the pluton margin, coarse-grained plagioclase is generally more calcic; however, a large degree of compositional overlap exists between samples. Compositional changes in the matrix (recystallized) plagioclase are more systematic, with a clear positive correlation between Ca content and proximity to the pluton margin (Fig. 35). The matrix plagioclase in sample B (~40 cm from the contact) has an average XCa of 0.47, up substantially from that of interior sample H (~XCa = 0.30). Higher Ca concentrations in the matrix plagioclase probably reflect the breakdown of calcic amphibole, the generally more calcic composition of the primary plagioclase grains that recrystallized/neocrystallized to form the matrix grains, and the possibility of preferential leaching of Na in comparison to Ca during metasomatic alteration (discussed below).
Symplectic intergrowths of fine-grained plagioclase, titanite and new biotite, and of myrmekite-like plagioclase and quartz, are observed locally replacing coarse grained (primary) biotite and plagioclase, respectively. The symplectic structure reflects simultaneous growth of all three minerals, and we infer that the reaction occurred in response to, or was assisted by, the deformation and enhanced element mobility. The composition of symplectic plagioclase intergrown with titanite is similar to that of the coarse grained (porphyroclastic) and matrix plagioclase (i.e., in the range of XCa = 0.30-0.40), but is locally of slightly different composition from its host grain (Fig. 37a). We infer that these symplectic intergrowths of fine-grained plagioclase, titanite and new biotite are produced by a reaction represented by the generalized equation:
and their widespread occurrence suggests that this type of reaction may be an important producer of fine-grained matrix plagioclase.
Recrystallized plagioclase in myrmekite-like intergrowths with quartz is substantially more calcic (XCa = 0.56 – 0.82) than the replaced plagioclase grain (XCa = 0.38-0.44; Fig. 37b), or any other plagioclase analysed in the sheared or unsheared tonalite. This microstructure is only observed in a thin (~1 cm) band in one of the strongly sheared samples (C), and may result from preferential leaching of Na during fluid fluxing or local Ca enrichment resulting from hornblende breakdown.
Figure 37. Backscattered electron image showing symplectic plagioclase, biotite and titanite replacing primary biotite.
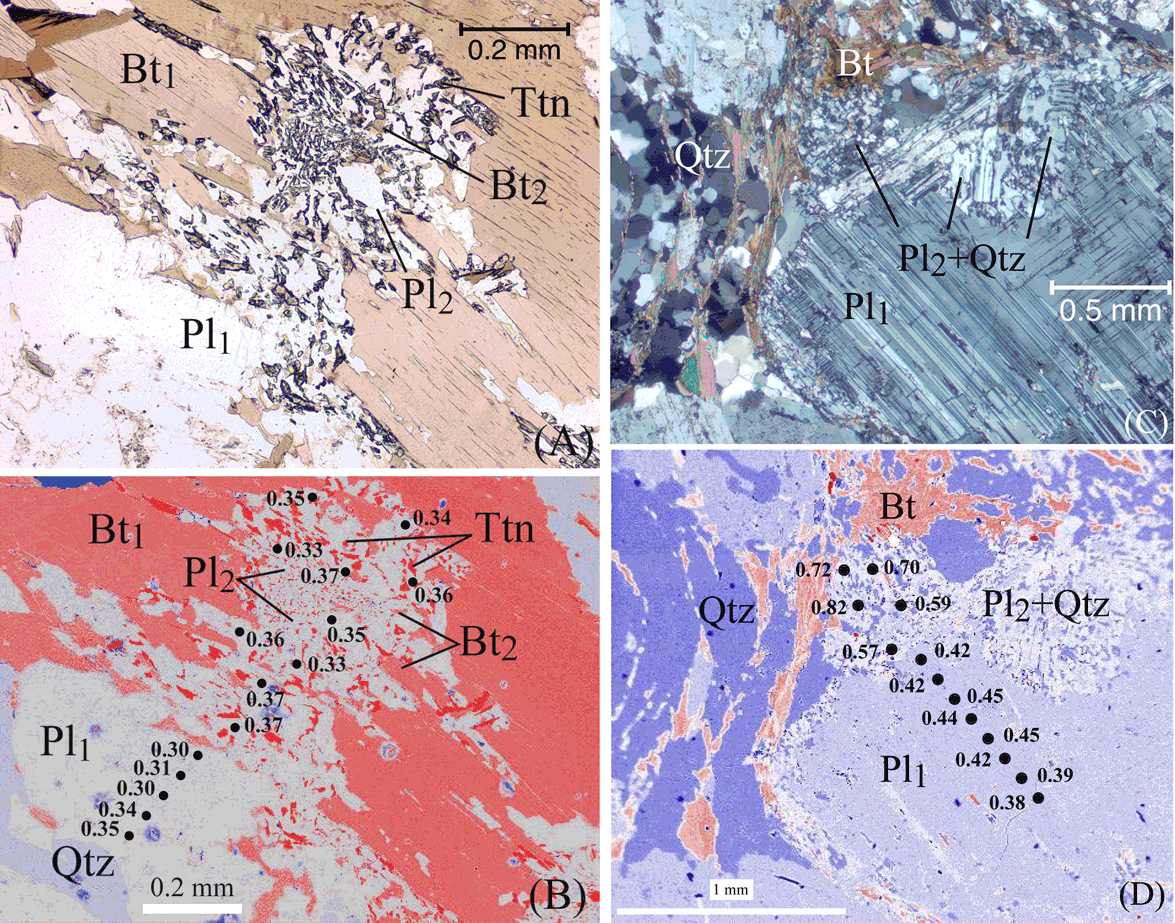
(A) Photomicrograph and (B) backscattered electron image showing symplectic intergrowths of fine-grained plagioclase (Pl2), biotite (Bt2) and titanite (Ttn) replacing a primary igneous biotite grain (Bt1). Points on backscatter image show plagioclase anorthite content. Photomicrograph plane-polarized light. (C) Photomicrograph and (D) backscattered electron image showing symplectic intergrowths of fine-grained plagioclase (Pl2) and quartz (Qtz) replacing a primary igneous plagioclase grain (Pl1). Points on backscatter image show plagioclase anorthite content. Photomicrograph crossed polars.
4.2.2. Biotite
As with plagioclase, systematic changes in biotite composition occur from the pluton interior into the shear zone. The biotite in the less deformed rocks is typically greenish brown, but much of the biotite that forms incipient new foliae in these rocks is red-brown. The red-brown biotite becomes more abundant with increased deformation and the biotite in the higher strain rocks is all red-brown. These observations suggest that the reactions responsible for the abundance of red-brown biotite in the mylonites were incipient in the least deformed rocks.
The most notable chemical difference accompanying this color change is a progressive increase in Al and decrease in Fe in biotite from higher strain rocks (Fig. 35; Table 3). The compositional changes coincide with syn-deformational production of new biotite grains through recrystallization of primary biotite grains, precipitation of fine-grained biotite in strain shadows adjacent to plagioclase clasts, and the replacement of hornblende by biotite via an inferred local reaction represented by the generalized equation:
We infer that the additional H2O and K needed to drive this reaction were provided primarily by infiltration of fluids through the shear zone, with some additional K available through the replacement of minor igneous K-feldspar by myrmekite. Depletion of Fe in the new biotite is consistent with the bulk decrease in Fe within the shear zone, whereas the Al enrichment could be due to its apparent immobility during metasomatism.
4.2.3. Hornblende
Magmatic hornblende is present throughout the Cerro de Costilla complex tonalite, but is conspicuously absent from the strongly sheared samples (A-C). This is presumably due to hornblende breakdown to biotite (Eq. 2). Primary hornblende grains fall within the edenite to magnesio-hornblende compositional range and commonly have moderate compositional zoning, with increasing Ca concentrations from core to rim. The hornblende mode is consistent across the tonalite interior, although the chemical composition changes. In sample D, fine-grained blue-green actinolitic hornblende is observed in the matrix foliation and on the margins of fragments. Lower Na, K, Fe, Ti, and Al, and higher Mg and Si concentrations are observed in both new (blue-green) and primary hornblende grains in sample D relative to H. These compositional changes are consistent with Fe-Mg exchange and the edenite substitution (exchange vector = Na-1Al-1Si□; Thompson et al., 1982), which describes the solid solution between Na and Al-rich edenite and actinolite. The compositional change could reflect re-equilibration at the lower crustal temperatures that occurred during deformation, as suggested by lower Ti concentrations (Otten, 1984, Pattison, 2003) or changes in the local effective bulk composition related to metasomatic mass exchange.
4.3. Hornblende – plagioclase thermometry
The compositions of coexisting hornblende and plagioclase grains within the foliated matrix of sample D were used to estimate the temperature of deformation (Table 4). Temperature estimates were calculated for various pressures using the hornblende-plagioclase thermometer of Holland and Blundy (1994), which is based on the edenite exchange equilibrium: Ed + 4Qtz = Tr + Ab. This thermometer was selected because of the observed change from primary edenitic hornblende to recrystallized actinolitic hornblende (e.g., Figs 20 and 21a) through edenite component exchange, the abundance of quartz in all samples (i.e., silica saturation), and its calibration down to 400°C. At 3 kbar, the calculated temperatures range from 490°C to 576°C, with an average of 538°C. Owing to the possibility of incomplete equilibration between each grain pair and the minor pressure dependence of the calculated values, we adopt a conservative uncertainty of + 75°C. It is possible that shearing in the hornblende-free marginal samples (A-C) continued during cooling, and therefore we consider ~540 + 75°C an upper limit on shear-zone temperature during deformation. This is consistent with the microstructural evidence, and in view of all evidence and data, we suggest that a reasonable bracket for the deformation temperature is 475 ± 50°C.
Figure . Table 4.
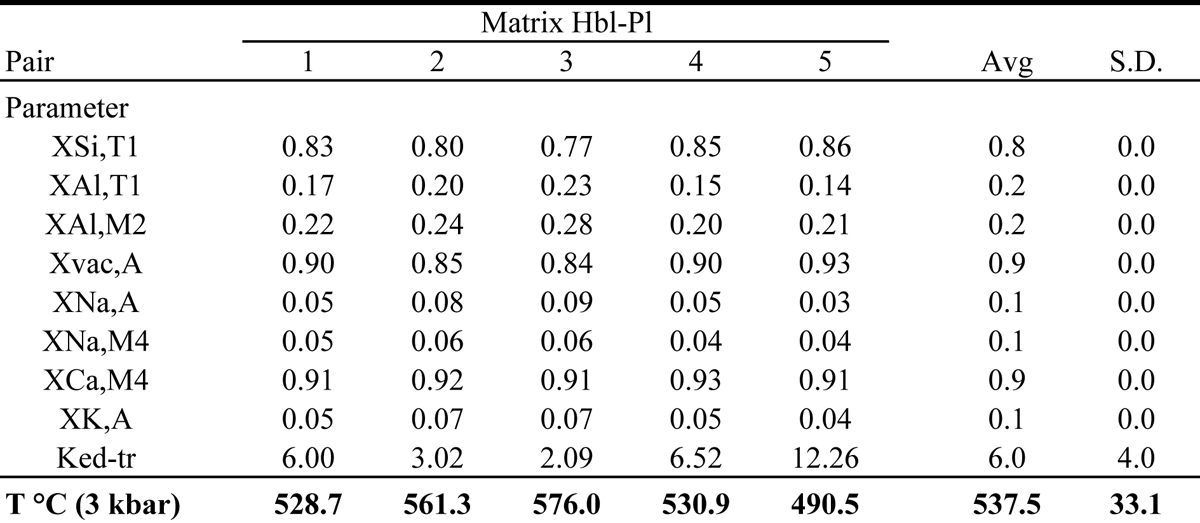
Parameters and calculated temperatures for Hornblende-Plagioclase pairs used for thermometry (after Holland and Blundy, 1994).