1. Introduction
The aim of this investigation is to evaluate the mechanical and chemical processes involved in the initiation and development of a mylonitic foliation in hornblende-biotite tonalite of the Cerro de Costilla complex, Baja California, México (Fig. 1). Many studies have shown that the development of a mylonitic foliation is accompanied by moderate to extreme grainsize reduction. Most of these studies have attempted to trace the progression from protomylonites with relatively large amounts of strain to mylonites or ultramylonites. This study explores the breakdown of a relatively homogeneous, undeformed protolith to a mylonite in which the phyllosilicates form an interconnected network of low-stress microshear zones. Stages of progressive fabric development are preserved across an approximately 28-m wide strain gradient at the margin of the complex, from essentially undeformed, coarse-grained tonalite to fine-grained, foliated mylonite at the wall-rock contact. We describe microstructural details of the transition, in which the interconnectivity of biotite and consequent foliation development were facilitated by fracture-assisted breakdown of a strong plagioclase framework, in addition to metasomatically-induced mineralogical changes. Whole-rock and mineral chemical data suggest a fluid infiltration front in the highest-strain rocks within 1 m of the host-rock contact, and we discuss the role of this inferred fluid in reactions that led to a modal increase in relatively weak minerals, namely biotite and quartz.
Figure 1. Geologic map showing the location of the Cerro de Costilla complex.
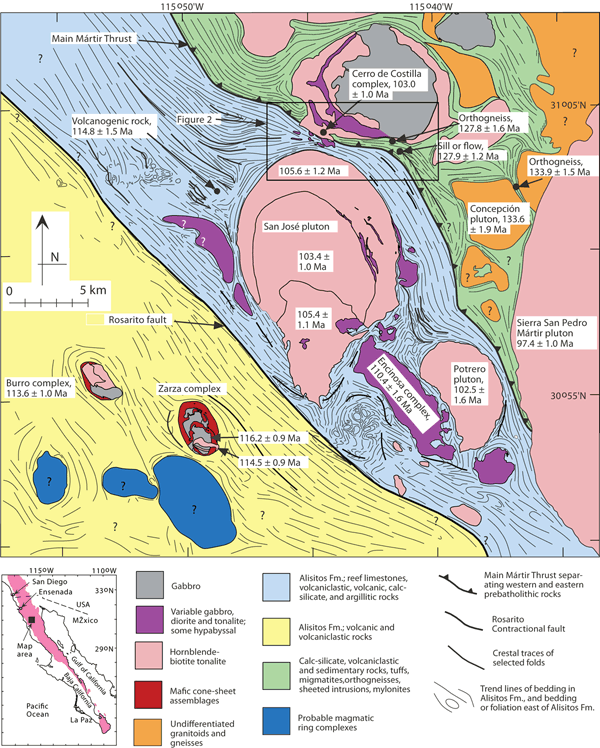
Geologic map of the San Jose pluton and surrounding region, from Johnson et al. (2003) with modifications from Melis (2006). The Cerro de Costilla complex is located in upper-center of the map. One age from the southern part of the complex is shown. All ages are from SHRIMP U-Pb zircon data reported in Johnson et al. (1999, 2003). See legend for geological information. Inset map at lower left shows the Baja California peninsula; the Peninsular Ranges batholith and adjacent pre-batholithic country rocks are shaded.
Grainsize reduction can arise from both brittle and ductile processes (e.g., Bell and Etheridge, 1973; White et al., 1980; Vernon et al., 1983; Segall and Simpson, 1986; Tullis and Yund, 1987; Goodwin and Wenk, 1995; Vernon et al., 2004; Ree et al., 2005), and is generally considered to be an important cause of strain localization in shear zones (e.g., White, 1979; Handy, 1989; Drury et al., 1991; Jin et al., 1998; Montesi and Hirth, 2003; Ree et al., 2005; Oesterling et al., 2007). In polymineralic rocks, an equally important mechanism for localization involves the interconnection of originally dispersed weak minerals, such as phyllosilicates (e.g., Kronenberg et al., 1990; Shea and Kronenberg, 1992, 1993; Mares and Kronenberg, 1993; Wintsch et al., 1995; Bos & Spiers, 2001; Johnson et al., 2004; Vernon et al., 2004; Neimeijer and Spiers, 2005; Holyoke & Tullis, 2006; Mariani et al., 2006; Marsh et al., in press). Coalescence of weak minerals into interconnected, anastomosing networks facilitates weakening, and may be particularly important at crustal levels coincident with the frictional-to-viscous transition (Wintsch et al., 1995; Stewart et al., 2000; Imber et al., 2001; Faulkner et al., 2003; Collettini and Holdsworth, 2004; Mariani et al., 2006; Marsh et al., in press), where mylonitic shear zones represent the roots of major faults and control the coupling of deformation between the middle and upper crust (e.g., Handy et al., 2007).
Theoretical and experimental work has shown that the bulk rheology of polymineralic rocks is dominated by the weakest mineral at volume fractions as low as approximately 15% (e.g., Price, 1982; Tharp, 1983; Jordan, 1987, 1988; Ross et al., 1987; Handy, 1990, 1994; Bons and Urai, 1994; Bons and Cox, 1994; Handy et al., 1999; Johnson et al., 2004; Holyoke & Tullis, 2006). However, the volume fraction of weak minerals, particularly phyllosilicates, commonly increases with increasing strain in shear zones, owing to syndeformational metamorphic hydration reactions (e.g., Vernon et al., 1983, Fitzgerald and Stunitz, 1993, Wintsch et al., 1995, Yonkee et al., 2003; Marsh et al., in press). Consequently, coupling of mechanical and chemical processes can lead to extreme weakening, which results in increased strain rates, additional grainsize reduction and enhanced reaction rates.
The mechanism of weak-phase linkage is typically difficult to identify in naturally deformed rocks, because the process initiates at extremely low strains, and the evidence is typically obliterated by progressive deformation and recrystallization/neocrystallization (e.g., Christiansen and Pollard, 1997; Bons and Jessell, 1999; Handy et al., 2001; Johnson et al., 2004). To find such evidence in polymineralic rocks, it is necessary to examine complete strain gradients. In addition, owing to the control exerted by preexisting layering, such as bedding or tectonic foliation, it is advantageous to study discrete shear zones in coarse-grained, non-foliated igneous rocks containing dispersed weak and strong minerals. Several studies have examined strain and fabric gradients in deformed granitoids involving strain-dependent coalescence of mica (e.g., Berthé et al., 1979; Simpson, 1985, 1985; Gapais, 1989; Lonka et al., 1998; Vernon et al., 2004; Johnson et al., 2004; Marsh et al., in press).
The two-part study of Vernon et al. (2004) and Johnson et al. (2004) is unusual, in that evidence for the microstructural mechanisms responsible for coalescence of originally dispersed biotite grains is clearly preserved. These authors found that linkage was initiated in undeformed tonalite by fracturing of the load-bearing plagioclase framework between intervening biotite grains, the fractures typically emanating from the tips of biotite grains, parallel to their (001) crystallographic faces. This was attributed to instantaneous strain-rate gradients among the different minerals (Johnson et al., 2004), as also argued by Tullis et al. (1991). Johnson et al. (2004) conducted numerical experiments to investigate foliation initiation, reporting a stress and strain-rate evolution strikingly similar to that found in later laboratory experiments on fine-grained biotite gneiss (Holyoke and Tullis, 2006). A similar process was identified in calcite-halite experiments (Jordan, 1987) and in low-temperature experiments on mica schists (Gottschalk et al., 1990; Shea and Kronenberg, 1992, 1993; Rawling et al., 2002).
The deformation gradient studied by Vernon et al. (2004) and Johnson et al. (2004) is not easily related to deformation experiments, because the rocks show evidence for the presence of a small amount of partial melt during the deformation. Thus, it is unclear what role fluid-enhanced embrittlement (e.g., Davidson et al., 1994; Connolly et al., 1997; Holyoke and Rushmer, 2002) may have played in fracturing and initiation of microshear zones. Therefore, one of our purposes here is to carefully examine the microstructural evidence for initiation of weak-phase linkages in a tonalite that shows no microstructural evidence that melt was present during the deformation, but instead reflects the results of brittle and crystal-plastic, solid-state processes, coupled with chemical reactions and metasomatic changes. The tonalite studied here is compositionally and modally nearly identical to the San José tonalite studied by Vernon et al. (2004) and Johnson et al. (2004). The deformation gradient in the San José tonalite was considered by these authors and Johnson et al. (2003) to have formed at high strain rates during emplacement and cooling of the magma. In contrast, the strain gradient evaluated here formed during greenschist-facies regional deformation. These two examples provide evidence for how strain localizes at different conditions, but for both, a key feature is coalescence of originally dispersed biotite grains into anastomosing microshear zones.