Mukhopadhyay, D. K. 2003. A melting mechanism for the Himalayan leucogranite. In: Singh, S. 2003. Granitoids of the Himalayan Collisional Belt. Journal of the Virtual Explorer, Electronic Edition, Volume 11, Paper 05, ISSN 1441-8142.
A melting mechanism for the Himalayan leucogranite
Abstract
A number of small and discontinuous leucogranite plutons of Miocene or younger ages occur in the Higher Himalayas. They have formed through post-collision partial melting of the Himalayan crust and are taken as a signature of the collision event. A mechanism of partial melting is described that is based on the relationship between depth-temperature-time (D-T-t) paths in a crust thickened due to intra-continental ovethrusting and lnK-dependent melting/solidus curves. It is suggested that deformation-induced fast segregation, ascent and emplcement of small magma pods formed at different depths and at different times can explain some of the features associated with the leucogranite plutons.
Keywords:
plutons, himalayan, melting, post-collision, leucogranite
Introduction
An important feature of the Himalayan orogenic belt, i.e., south of the Indus-Tsangpo Suture Zone (ITSZ), is the occurrence of a number of small and discontinuous leucogranite plutons. These plutons occur in two conspicuous linear belts (Fig. 1): at or near the contact between the medium- to high-grade metamorphic rocks of the High Himalaya Crystalline Zone (HHCZ) and the overlying Palaeozoic sedimentary sequence belonging to the High Himalaya Sedimentary Zone (HHSZ), and within the HHSZ. It is now well established that these leucogranite plutons have formed due to partial melting of the Himalayan crust during the Tertiary period, i.e., after the terminal collision between the Indian and the Eurasian plates. Consequently, these plutons have been taken to represent a signature of the collision event by some workers (e.g., Le Fort, 1981). LeFort (1981) and Lefort et al. (1987) gave a model for the generation and emplacement of the Himalayan leucogranites (Fig. 2). According to this model, continental-scale thrusting along the Main Central Thrust (MCT) brought the hot portion of the deep continental crust to lie on top of the Lesser Himalaya Zone (LHZ, Midland Formation in Nepal). This induced synkinematic metamorphism of the rocks of the LHZ that released large amount of H2O-CO2 fluid through decarbonation and dehydration reactions. In turn, this fluid rose above the MCT lowering the solidus in the hot HHCZ and induced partial anatexis and produced melts close to minimum melt composition. The melt rose through the metamorphic piles along giant dykes and sills and emplaced at the contact between the HHCZ and HHSZ or within the HHSZ. Detailed mapping and traverses through rather inhospitable terrains by later workers (e.g., Scaillet et al. 1995; Searle et al. 1997, 1999; Searle 1999) have shown that many of the Himalayan leucogranite plutons are indeed underlain and fed by giant dyke and sill complexes. Therefore, the model envisaged by Le Fort (op. cit.) for the emplacement of leucogranitic magma seems to be well documented from different areas. However, the Le Fort's model of the mechanism of partial melting in the Himalayan crust needs modification for several reasons. Nowhere along the entire length of the Himalayas there is any evidence of partial melting in the rocks occurring in the immediate hangingwall of the MCT. Further, the footwall of the MCT is occupied by sedimentary or very low grade metamorphic rocks that do not appear to have given off large amount of fluid. Experimental studies on the rocks from the hangingwall of the MCT show that only fluid absent melting generates melts that are identical in composition to the Himalayan leucogranites (Patiño Douce and Harris 1998). Finally, the rocks in the hangingwall in a crust thickened by overthrusting initially cools as the heat is conducted to the footwall from the hangingwall and it may take a few tens of m.y. for the hangingwall to regain its initial temperature (Oxburgh and Turcotte 1974; England and Thomson 1984).
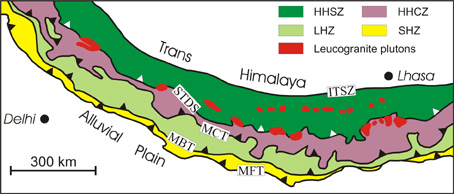
A possible melting mechanism for the Himalayan leucogranites, based on the transient geotherms and depth-temperature-time (D-T-t) paths of rocks in an eroding over-thickened crust (Mukhopadhyay 1999), is desribed here. It is emphasized that the calculated thermal structure presented here is for demonstration of the melting mechanism only. Detailed thermal modelling is not the primary objective of this article.
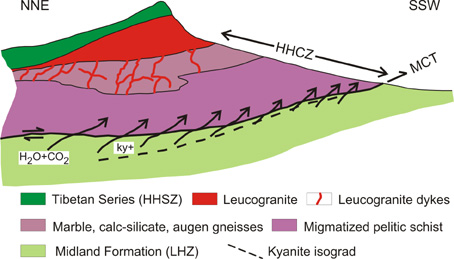
Tectono-thermal model
Following the continent-continent collision, the continued convergence resulted in the frontal part of the Indian plate to get thrusted back onto itself along a gently dipping detachment (Fig. 3; Seeber et al. 1981; Hirn 1984; Ni and Barazangi 1984). This detachment is referred to as the Main Himalayan Thrust (MHT, Zhao et al. 1993). The detachment extends from the foreland to the Higher and Tethys Himalayas (Zhao et al. 1993) resulting in the doubling of the Himalayan crust to about 70-80 km. The Indian continental crust remains intact under the detachment at least up to the middle of the Tethys Himalaya. However, it is uncertain how far north the detachment extends, and in particular if it extends beyond the ITSZ or not (Makovsky et al. 1996). It has been suggested that this detachment dips at about 4-7° towards north below the frontal part of the orogenic belt (Seeber et al. 1981; Mukhopadhyay and Mishra 1999). The dip of this detachment below the Tethys Himalaya has been estimated to be about 9±2° (Zhao et al. 1993; Makovsky et al. 1996). With this amount of dip, the detachment reaches a mid-crustal depth of about 30-40 km below the Higher and Tethys Himalayas. A tectonic model with a horizontal thrust at a depth of about 30 km has been taken for the purpose of calculating thermal structure in the Himalayas. Given the uncertainties in the heat flow parameters, neglect of a few degrees dip of the detachment should not add significant errors in interpretation. Further, the thrusting velocities are much faster than the rate of heat conduction within the crust. Therefore, the geotherms at any given time since thrusting can be calculated using one-dimensional heat-conducting equation for a vertically moving medium (Carslaw and Jeager 1959):
(1)
This approach has been taken by many workers in the past (e.g. Molnar et al. 1983; Moore and England 2001). The main difficulty in the applicability of heat conducting equations, such as eq. 1, for the Himalayan orogen is that values of too many heat-flow parameters are unknown and only a range of possible values can be assumed leading to a range of possible interpretations. For example, Molnar et al. (1983) used eq. 1 to show that various combinations of heat flow parameters can generate partial melting in the Himalayan crust and more than 1 kbar shear stress would be required to melt rocks at or immediately above the detachment. Molnar et al. (1983) also suggested that it is possible to generate granitic melt from below the detachment by a combination of radioactive heating in the crust and heat supplied by the mantle.
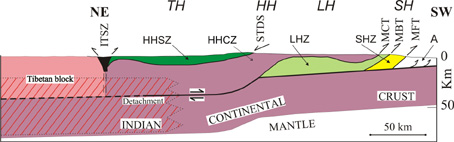
Melting mechanism
Generation of leucogranitic magma through partial melting within the crust is a complex process and depends on several factors but it is imperative that the so-called 'fertile' rocks must be heated above the appropriate solidus/melting curves in order to trigger melting. In other words, melting should occur at depths below the intersection between the geotherm and the solidus/melting curve.
In overthrust terrains the geotherm remains strongly transient for several tens millions of years following crustal thickening. Immediately after crustal thickening the geotherm is perturbed as a consequence of fast heat advection through thrusting as compared to the rate of heat conduction. This perturbation decays with time but remains strongly transient for few tens of m.y. after thrusting because the rocks are, in general, are bad conductors of heat. This should be true no matter what the values of heat-flow parameters are or whether the thermal structures are modelled using one-dimensional heat conducting equation or mathematically more involved two- or three-dimensional models. In Fig. 4 geotherms at 5, 10, 20, 30 40, and 50 m.y. after thrusting are shown. The geotherms are calculated using heat-flow parameters as given in the caption to Fig. 4 and using mathematical solutions to eq. 1 as given in Molnar et al. (1983). The geotherms are calculated up to "50 m.y." because that is the approximate time for the terminal collision in the Himalayas (Klootwijk et al. 1992). It is unknown when the detachment formed, if it formed immediately after collision then the curve labelled "50 m.y." should represent the approximate present-day geotherm. If the detachment formed sometime after the collision, then the present-day geotherm should move to the lower temperature side accordingly.
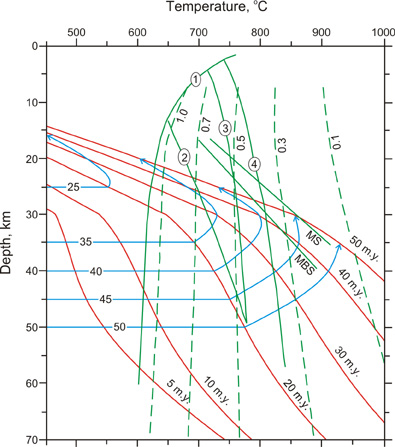
Sometime after the crustal thickening due to overthrusting, the rocks at depth will be progressively unroofed because of erosion at the surface. Since the geotherms are transient, a vertically moving rock will not follow a particular geotherm. This is illustrated in Fig. 4 through a series of depth-temperature-time (D-T-t) paths for rocks initially at different depths. The D-T-t paths have been calculated assuming no erosion for the first 20 m.y. followed by long time average erosion rate of 0.5 mm/year (cf. Thompson 1981). As the rocks in the footwall move towards the surface, they first get heated up then cool giving rise to counterclockwise path in D-T-t space. But the rocks in the hangingwall first cool and then follow a counterclockwise D-T-t path.
Fig. 4 also shows a few possible melting/solidus curves that may be appropriate for the rocks in the HHCZ. The locations of these curves as shown are not unique because they dependent on distribution coefficient (lnK). The dominant rock types in the HHCZ are granitic gneisses and metapelites and both can be considerd to be 'fertile' rock for the generation of granitic melt. However, their mineralogical as well as bulk compositions may vary both along strike and depth. Consequently, melting reactions can be different at different locations in the Himalayas.
It is to be noted that the transient geotherms, the D-T-t paths and the melting/solidus curves can all be varied by assuming different set of values for the set of physical parameters used for calculations. However, the geometric relation between them, as shown in Fig. 4, should be approximately similar. The following discussion on a possible melting mechanism is based on the relation between these curves. It is not the purpose of this article to constrain the depth, temperature or time of melting for any specific leucogranite pluton in the Himalayas.
Let us consider the H2O-saturated pelite solidus (curve 1, Fig. 4) and the D-T-t paths for rock which was originally at a depth of 35 km. Partial melting in this rock can be initiated when the D-T-t paths cross over to the higher temperature side of curve 1. However, melting will stop when the D-T-t path cross over to the lower temperature side with progress of time. Also, it is possible that the lnK of the melting reaction may change through, for example, one or more of the phases taking part in other reactions operating simultaneously. If the lnK changes in such a way that the solidus curve shifts to the higher temperature side, the melting may cease. Finally, D-T-t paths of rocks originally at different depths together with pelite solidus curve suggest that the minimum depth of melting becomes progressively shallow with progress of time.
Consideration of D-T-t paths and aH2O-dependent granite solidus curves (Fig. 4) also leads to similar generalization. At deep crustal levels the Precambrian crystalline rocks are not expected to be pervasively saturated with H2OO and XH2O may be different at different depths. So at different depths different granite solidus (i.e., with different aH2O) may be appropriate. This leads to the possibility that the granite may melt at different depths at different time and temperature. Further, the little amount of H2O that may be present in pores and along grain boundaries may help to produce a melt. But as the melt forms, H2O would preferentially partition into the melt driving the granite solidus to the higher temperature side leading to cessation of melting. As a consequence the total amount of melt formed at any depth should be small and likely to be leucocratic.
Discussion and conclusions
An important consequence of the melting mechanism described above is that so long as 'fertile' rocks are present, partial melting can occur at different depths and at different times. Partial melting need not be confined to any particular stratigraphic horizon or tectonic plane (e.g., MCT) as postulated earlier by some workers (e.g., LeFort et al. 1987) (Fig. 2). At any particular locality, the depth and time of melting will depend on the exact shape of the D-T-t paths and the appropriate -lnK-dependent melting/solidus curve. As mentioned earlier, the D-T-t paths may vary along the orogen depending on the heat-flow parameters and erosion rates. In this scenario, partial melting can affect wide swath of the thickened Himalayan crust, both along and across the orogen as well as at different depths. Depending on the values of heat-flow parameters, it is also possible for the transient geotherms to have steeper gradient than the initial (i.e., at the time of thrusting) geothermal gradient. Consequently, partial melting and emplacement of granitic magma may be continuing to the present day. Very young ages obtained from some of the plutons (e.g., Zeitler and Chamberlain 1991) and high surface heat flow in Tibetan plateau (Francheteau et al. 1989) supports this contention. This is also in conformity with the deduction from seismic data of a partial molten crust in southern Tibet (Nelson et al. 1996; Kind et al. 1996).
The extraction of the small pods magma and their buoyancy-induced ascent and emplacement would be difficult and agonizingly slow because of high viscosity. However, since the collision at about 50 Ma, the Himalayan rocks are being continuously deformed due to continued convergence. This deformation expresses itself by a large number of thrusts that slice up the Himalayan rock sequences to form a crustal stacking wedge. Besides, the source rock for partial melting belong to the HHCZ which is characterized by extensive development of deformation related foliations. It is therefore reasonable to postulate that deformation may have played an important role in magma extraction, ascent and emplacement (cf. Brown 1994). This is supported by the fact that the Himalayan leucogranites are underlain by feeder dykes along which rates of magma flow was very fast (Scaillet et al. 1996).
An interesting feature of the Himalayan leucogranites is extreme heterogeneity in Sr isotope ratios, even in the scale of outcrop, such that reliable Rb-Sr isotopic ages are difficult to obtain (e.g., Daniel et al. 1987; Stern et al. 1989). The melting mechanism detailed here generates small pods of magma at different locations in the crust at different times. Each of the pods will have it's own unique Sr isotope ratios depending on the isotope ratios of the source rock undergoing partial melting. The deformation-induced fast segregation, ascent and emplacement together with slow rates of homogenization of isotope ratios may help the small magma pods to retain the original isotope ratio (Mukhopadhyay 2001). Since the time scale of Himalayan orogeny is small (<50 Ma), the measured Sr isotope ratios are close to the original ratios acquired at the time of melting. This adequately explains chaotic variations in Sr isotope ratios in most Himalayan leucogranite plutons.
References
- Brown, M. (1994) The generation, segregation and emplacement of granite magma: the migmatite-to-crustally-derived granite connection in thickned orogens. Earth Sci. Rev., 36:83-130.
- Carslaw, H. S. and Jeager, J. C. (1959) Conduction of Heat in Solids. Oxford University Press, 510p.
- Deniel, C., Vidal, P., Fernandez, A., Le Fort, P., and Peucat, J-J. (1987) Isotopic study of the Manaslu granite (Himalaya, Nepal): Inferences on the age and source of Himalayan Leucogranites. Contrib. Mineral. Petrol., 96:78-92.
- Dietrich, V. and Gansser, A. (1981) The leucogranites of the Bhutan Himalaya (Crustal anatexis versus mantle melting). Schweiz. Mineral. Petrogr. Mitt., 61177-202.
- Ebadi, A. and Johannes, W. (1991) Beginning of melting and composition of first melts in the system Qz-Ab-Or-H2O-CO2. Contrib. Mineral. Petrol., 106: 286-295.
- England, P, C, and Thompson, A. B. (1984) Pressure-temperature-time paths of regional metamorphism of the continental crust, I, Heat transfer during the evolution of regions of thickened continental crust. Jour. Petrol., 25:894-928.
- Francheteau, J. and 7 others (1984) High heat flow in southern Tibet Nature, 307:32-36. Hirn. A, and 11 others (1984) Crustal structure and variability of the Himalayan border of Tibet. Nature, 307: 23-25.
- Kind, R. and project INDEPTH team (1996) Evidence from earthquake data for a partially molten crustal layer in southern Tibet. Science, 274:1692-1694.
- Klootwijk, C. T., Gee, J. S., Peirce, J. W., Smith, G. M. and McFadden, P. L. (1992) An early India-Asia contact: paleomagnetic constraints from Ninetyeast Ridge, ODP Leg 121. Geology, 20:395-398.
- Le Fort, P. (1981) Manaslu leucogranite: a collision signature of the Himalaya. A model for its genesis and emplacement. Jour. Geophys. Res., 86:10545-10568.
- Le Fort, P., Cuney, M., Deniel, C., France-Lanord, C., Sheppard, S. M. F., Upreti, B. N. and Vidal, P. (1987) Crustal generation of Himalayan leucogranites. Tectonophysics, 134: 39-57.
- Makovsky, Y., Klemperer, S. L., Liyan, H. and Deyuan, L. (1996) Structural elements of the southern Tethyan Himalaya crust from wide-angle seismic data. Tectonics, 15:997-1005.
- Molnar, P., Chen, W-P. and Padovani, E. (1983) Calculated temperatures in overthrust terranes and possible combination of heat sources responsible for the Tertiary granites in the greater Himalaya. Jour. Geophys. Res., 88:6415-6429.
- Mukhopadhyay, D. K. (2001) Extreme heterogeneity in Sr isotope systematic in the Himalayan leucogranites: A possible mechanism of partial melting based on thermal modeling. Proc. Indian Acad. Sci. (Earth Planet. Sci.), 110:161-169.
- Mukhopadhyay, D. K. and Mishra, P. (1999) A balanced cross section across the Himalayan foreland belt, the Punjab and Himachal foothills: A reinterpretation of structural styles and evolution. Proc. Indian Acad. Sci. (Earth Planet. Sci.), 108:189-205.
- Nelson, K. D. and and project INDEPTH team (1996) Partially molten middle crust beneath southern Tibet: Synthesis of project INDEPTH results. Science, 274:1684-1688.
- Ni, J. and Barazangi, M. (1984) Seismotectonics of the Himalayan collision zone: geometry of the underthrusting beneath the Himalaya. Jour. Geophys. Res., 89:1147-1163.
- Oxburgh, E. R. and Turcotte, D. (1974) Thermal gradients and regional metamorphism in overthrust terrains with special reference to eastern Alps. Schweiz. Mineral. Petrogr. Mitt., 54:641-662.
- Scaillet, B., Holtz, F., Pichavant, and Schmidt, M. (1996) Viscosity of Himalayan leucogranites: Implications for mechanisms of granitic magma ascent. Jour. Geophys. Res., 101:27691-27699.
- Scaillet B, Pecher A, Rochette P and Champenois M 1995 The Gangotri granite (Garhwal Himalaya): laccolithic emplacement in an extending collisional belt; Jour. Geophys. Res. 100 585-607
- Sclatter, J. G., Jaupart, C. and Galson, D. (1980) The heat flow through oceanic and continental crust and heat loss of the earth. Rev. Geophys. Space Phys., 18:269-311.
- Searle, M. P. (1999) Emplacement of Himalayan leucogranites by magama injection along giant sill complexes: examples from Cho Oyu, Gyachung and Everest leucogranites (Nepal Himalaya). Jour. Asian Earth Sci., 17:773-783.
- Searle, M. P., Parrish, R. R., Hidges, K. V., Hurford, A., Ayres, M. W. and Whitehouse, M. J. (1997) Shisha Pagma leucogranite, south Tibetan Himalaya: field relations, geochemistry, age, origin and emplacement. Jour. Geol., 105:295-317.
- Searle M. P., Noble, S. R., Hurford, A. J. and Rex, D. C. (1999) Age of crustal melting, emplacement and exhumation history of the Shivling leucogranite, Garhwal Himalaya. Geol. Mag., 136:513-525.
- Seeber, L., Ambruster, J. G. and Quittmeyer, R. C. (1981) Seismicity and continental subduction in the Himalayan arc. In, Zagros, Hindu-Kush, Himalaya Geodynamic Evolution, (eds.) Gupta, H. K. and Delany, F. M., Geodynamic Series (AGU, Washington), 3:215-242.
- Spear, F. S. (1993) Metamorphic phase equilibria and pressure-temperature-time paths. Min. Soc. Amer. Monograph, 799p.
- Stern, C. R., Kligfield, R., Schelling, D., Virdim N. S., Futa, K. and Peterman, Z. E. (1989) The Bhagirathi leucogranite of High Himalaya (Garhwal, India): age, petrogenesis, and tectonic implications. Geol. Soc. Am. Sp. Paper, 232:33-45.
- Thompson, A. B. (1981) The pressure-temperature (P,T) plane viewed by geophysicists and petrologists. Terra Cognita, 1:11-20.
- Zhao, W., Nelson, K. D. and project INDEPTH team (1993) Deep seismic reflection evidence for continental underthrusting beneath southern Tibet. Nature, 366:557-559.
- Zeitler, P. K. and Chamberlain, C. P. (1991) Petrogenetic and tectonic significance of young leucogranites from the northwestern Himalaya, Pakistan. Tectonics, 10:729-741.