Singh, S., Mukherjee, P. K., Jain, A. K., Khanna, P. P., Saini, N. K. and Kumar, R. 2003. Source characterization and possible emplacement mechanism of collision-related Gangotri Leucogranite along Bhagirathi Valley, NW-Himalaya. In: Singh, S. 2003. Granitoids of the Himalayan Collisional Belt. Journal of the Virtual Explorer, Electronic Edition, Volume 11, Paper 06, ISSN 1441-8142.
Source characterization and possible emplacement mechanism of collision-related Gangotri Leucogranite along Bhagirathi Valley, NW-Himalaya
Abstract
The Gangotri leucogranite is one of the largest bodies in the Higher Himalayan Leucogranite (HHL) belt. They occur as en-echelon lenses within the Pan-African S-type granitoid body. The possible source of proximal high-grade metasedimentary rocks and migmatites has yet to be established due to contrasting geochemistry. However, the observed geochemical linearity in binary variation diagrams and the sub-parallel nature of REE and spider-diagram patterns and other trace element data for the Pan-African body and tourmaline-bearing leucogranite suggest that the Pan-African body is the best potential source candidate. The separation of a small fraction of felsic melt from the source due to high viscosity is attributed to compactional or shearing stress during the syn-collisional event of Himalayan orogeny. The geochemical signatures also suggest that the leucogranites were produced by a lower degree of partial melting probably at shallower depth and low temperature.
Keywords:
Himalayan granites, collisional magmatism, geochemistry, geochronology, Higher Himalayan Leucogranite belt
Introduction
The Himalayan orogenic belt contains various granitoids, which have been assigned ages ranging from Proterozoic to Late Cenozoic by earlier workers, mainly based on field relationships, nature of xenoliths, degree of metamorphism, petrographical similarities, structural trends, etc., prior to radiometric age dating (McMahon, 1884; Greisbach, 1893; Auden, 1935; Wadia, 1928, 1957; and others). Workers have also observed that most of the peaks with heights over 7,000 m are made up of gneiss, granite or crystalline rocks in the Higher Himalaya and beyond (Odell, 1983). The Himalayan granitoids can be broadly classified into two main types in relation to the Himalayan orogeny: (i) pre-collisional granite/gneiss and (ii) syn-to post-collisional leucogranite. Geochronological data, reported on a variety of granitoids and minerals using different radioactive systems, have constrained the age of emplacement, cooling and exhumation history (Pande, 1999; Jain et al., 2000; Hodges, 2000; Singh, 2001 and references therein). Age data from the granitoids of the Himalaya reveal distinct episodes of magmatic activity around 2100-1800 Ma, 1200-1000 Ma, 600-400 Ma, 100-50 Ma and 25-15Ma (Singh, 2001).
The Himalayan granitoids have varied geographical distribution in along linear belts parallel to the Himalayan orogen. Large granitoid bodies occur in almost all the tectonic units, except the Sub-Himalaya. On the basis of the geographical distribution, granitic plutons constitute five major belts in the Himalaya and adjoining Karakoram (Le Fort, 1988). From north to south, these belts include (Fig. 1) namely; Karakoram Axial Batholith, Trans-Himalayan Batholith, Northern Himalayan Granite Belt, Granitoids of the Higher Himalayan Crystallines (HHC) Belt, and Lesser Himalayan Granite Belt
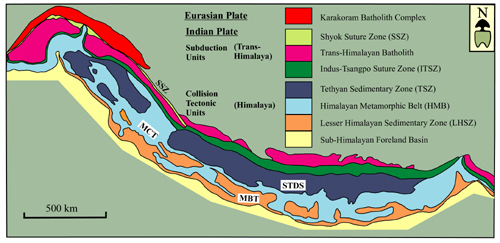
The Higher Himalayan Leucogranite (HHL), form the part of granitoids of the Higher Himalayan Crystallines (HHC), is of great interest to geologists working in orogenic belts and provides important information about the tectonic and metamorphic processes during orogenesis. Such leucogranites also occur in other regions of Western Europe, SE-Asia and Western United States, but their volume is relatively small. The Himalayan leucogranite, occurring as a belt, is probably the best exposed and among the most intensively studied granites so far. The High Himalayan Leucogranite (HHL) forms a discontinuous belt for about 1500 km from Pakistan to Bhutan (Fig. 2), having numerous intrusions of varying dimensions (Debon et al., 1986). These occur as sills laccoliths and are intruded either within the HHC or near its interface with the overlying unmetamorphosed to weakly metamorphosed Tethyan Sedimentary Zone (TSZ), where a major structural break of Miocene age has been postulated (Debon et al. 1986; Le Fort, 1986; Searle and Rex, 1989; Hodges et al., 1992).
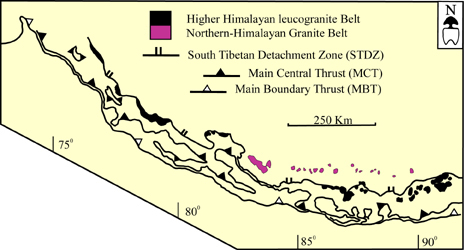
This contribution is an attempt to constrain the source characterization and possible emplacement mechanism of the collision-related Gangotri Leucogranite along Bhagirathi Valley, NW-Himalaya, India.
Geological Framework
The Gangotri Granite is the western end of the Badrinath Granite as one of the largest bodies of the High Himalayan Leucogranite (HHL) belt in the Garhwal Himalaya. It is exposed along the upper reaches of the Bhagirathi River around the Gangotri glacier region, including the peaks of Thalay Sagar (6904 m), Bhagirathi (6856 m), Meru Parbat (6672 m), Shivling (6543 m) and Bhigupanth (6044 m). The granite was first described by Heim and Gansser (1939) from the Higher Himalaya along upper Alaknanda Valley near Badrinath. Later, Auden (1949) described fine grained tourmaline+muscovite+biotite +garnet granite from the upper Bhagirathi Valley and termed it as the Gangotri Granite.
In the Gangotri region, two types of granitoids have been described from the upper reaches of the Bhagirathi River (Fig. 3; Pant, 1986; Pant and Dave, 1994). The Gangotri Granite is made up of several lenses or small leucogranite plutons, which are 1.5-2 km thick and 4-5 km long (Scaillet et al., 1990; Searle et al., 1993, 1999) in contrast to a single large Manaslu pluton. These lenses intrude either the metamorphosed base of the Tethyan Sedimentary Zone (Martoli Formation), the Harsil Formation (Pant, 1989), or occur in a large body of two-mica megacrystic granite, which has been named as the Bhaironghati Granite (Pant, 1989). The latter also intrudes the quartzo-feldspathic gneiss and schist of the HHC below and the Martoli Formation at the top. The emplacement of a number of en-echelon lenses of granite bodies around Gangotri and further southeast appears to have taken place along an extensional shear zone near the top of the HHC (Searle et al., 1993).
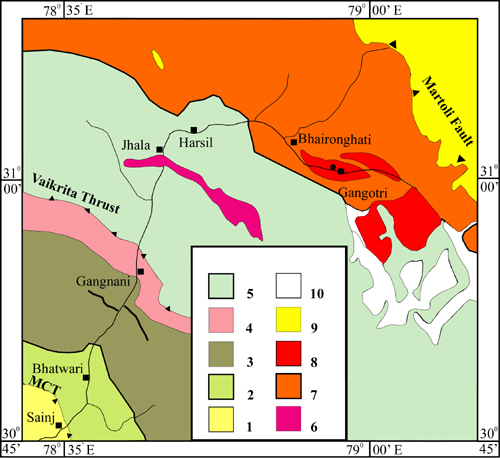
Stern et al. (1989) conducted Rb-Sr measurements on the whole rock and mineral separates (two-feldspar, muscovite and tourmaline) from the Gangotri Granite, and observed significant scattering on the isochron diagram. The Gangotri Granite, also known as the Badrinath body, is composed of several bodies. Deep incision by the Bhagirathi River provides different exposures of these bodies at Shivling, Bhagirathi, Meru and Thalay Sagar peaks. The whole rock analyses of leucogranite have yielded a 5-point Rb-Sr isochron of 21.1±0.9 Ma (Stern et al., 1989). However, Th-Pb monazite gave an age of 22.4±0.5 Ma for the Gangotri and 21.9±0.5 Ma age for the Shivling leucogranites (Harrison et al., 1997b). Searle et al. (1999) obtained a U-Pb monazite age of 23.0±0.2 Ma for the Shivling body. All these ages have been interpreted as the recrystallization age for the Gangotri-Badrinath group of leucogranites in the Garhwal Himalaya. Cooling ages for the Gangotri Granite were found to be 17.9±0.1 Ma using 40Ar/39Ar method for muscovite and between 2.41±0.52 Ma and 1.48±0.60 Ma by fission track dating of zircon and apatite, respectively, for a temperature range between 250±500C and 110±100C (Sorkhabi et al., 1996, 1999).
Field SettingsThe Gangotri Granite intrudes both the Bhaironghati Granite and the Martoli Formation of the Tethyan Sedimentary Zone. Field observations indicate that a network of aplite-pegmatite dykes and the Gangotri Granite cross-cut the foliation of the biotite granite gneiss and metasedimentary host rocks. The contacts between the two granites are always sharp. However, leucogranite lenses can be traced for a few kilometres along cliffs of biotite granite/gneiss. Overall, these observations indicate a late-to post-metamorphic emplacement of the Gangotri Granite.
Along the main road section from Jangla to Gangotri and beyond, two distinct granites occur (i.e. the biotite granite/gneiss and tourmaline-bearing leucogranite). At the Jangla Bridge, biotite granite is deformed into a medium to coarse-grained granite gneiss at the lower structural levels, close to the contact with the Harsil metamorphics. It extends along the northern slopes of the Bhagirathi Valley between Sian Gad, Harsil and Mukba. This granite is strongly foliated to granite gneiss at the contact with the metamorphics, but the main body remains undeformed and is medium to coarse grained and equigranular with occasional xenoliths. Numerous veins of tourmaline-muscovite leucogranite and garnet-beryl-tourmaline pegmatite intrude the biotite granite and the metamorphics. The Bhairoghati Granite contains mainly quartz+ orthoclase+ perthite+ microcline+ plagioclase+ biotite±muscovite ±zircon±apatite± epidote. Feldspar margins are sheared recrystallized and foliated and, at times, developed myrmekite.
The structure of the Gangotri Granite is usually characterized by a very weak foliation, defined by mica and tourmaline. Near the contacts, the foliation grades into layering which includes a few cm-thick bands of tourmaline. Two kinds of xenoliths are present within the leucogranite. The most abundant ones, always located near the contacts, are angular pieces of the host rock, which are often up to several meters in length. This results into the subparallel upper contact with the overlying Martoli black slates, which are metamorphosed into the staurolite-grade around Bhagirathi and Thalay Sagar peaks (Stern et al., 1989).
The temple at Gangotri is built on the tourmaline-bearing leucogranite; the leucogranite is fine-grained, massive, northerly-dipping sheet-like lens, with tourmaline crystals generally oriented perpendicular to the granite body wall. It is dissected by a set of steep to vertical joints through which the Bhagirathi River and the Jadh Ganga cut extremely narrow gorges.
The Gangotri Granite is homogenous, and equigranular and hypidiomorphic. The body contains quartz+ feldspar+ muscovite+ tourmaline± opaques± apatite± biotite± monazite± zircon± garnet. Both tourmaline and muscovite are of magmatic origin. The accessory minerals are randomly oriented in an overall quartz-orthoclase matrix. The inferred crystallization sequence is characterized by the early appearance of plagioclase, quartz and biotite, and late crystallization of the k-feldspar. It appears to be in the near-minimum melt composition, as their plot cluster around the invariant point in kf-Q-Plag-H2O diagram (Johannes and Holtz, 1990).
Geochemistry
Twenty nine granitoid samples from the Bhairoghati Granite and Gangotri Granite have been analysed for their major and trace elements using standard WD-XRF technique. Seven samples among them, collected along Bhagirathi and Jadh Ganga valley (Fig. 4) were also critically chosen and analysed for their REE abundance by ICP-AES technique (Rathi et.al. 1996). Analytical procedures, sample preparation and instrumental conditions are detailed in Saini et al. (2002) for XRF analysis and Rathi et al. (1996) for REE analysis.
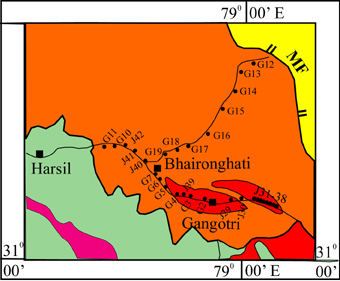
The Gangotri Leucogranite (LG) shows a rather restricted range of major and trace elemental abundance compared to the Bhairoghati Granite (BG), also referred to as biotite granite (Table 1 & 2). Analyses of major elements of these granitoids indicate that these are peraluminous with an Al2O3/Na2O+K2O+CaO ratio >1.1(Zen 1986, Chappel and White, 1992). Major elemental abundances show strong correlations with SiO2 notably having a negative trend for CaO representing assimilation and early crystallization of calcic plagioclase in these granites (Fig. 5 a). The biotite granite has higher FeO+ MgO, TiO2 and Ni content than the leucogranite; the overall decreasing trend of FeO (t) and MgO as SiO2 increases suggests high fractionation of mafic minerals like biotite, magnetite, etc. (Figs. 5 b, c). Low abundance and less spread of these elements in the leucogranite indicate lower abundance of mafic minerals and their insignificant role in fractional crystallisation. There is no systematic pattern of P2O5 with SiO2, indicating formation of apatite at all stages of crystallization in biotite granite, however, the range of P2O5 in leucogranite is small (Fig. 5 d). There is an apparent negative correlation between TiO2 and SiO2 in biotite granite indicating the role of Fe-Ti oxide and biotite in the fractionation process. (Fig.5 e). However, there is no distinct trend between Al2O3 and SiO2, except a feeble negative correlation in leucogranite indicating formation of feldspar (Fig. 5 f). The LG are strongly enriched in SiO2, alkalies and alumina and depleted in CaO. There is a positive correlation of SiO2 and Na2O mainly in the leucogranite (Fig. 5 g). No possible correlation exists between MnO and SiO2 in both the granitoids (Fig. 5 h). Several geochemical trends were earlier noted by Scaillet et al. (1990) from main body of the Gangotri Granite where an increase in Na2O, Rb, Sr, U, B and F, and a decrease in K2O, Fe2O3, TiO2, Sr, Ba, Zr, REE and Th were noticed with a decrease in CaO content. These variations can be best explained by the fractionation process of appropriate phases. Experimental solubility models for zircon and monazite in felsic melt support a magmatic origin for these two accessory phases as well (Scaillet et al., 1990). A tight correlation between Zr-TiO2 strongly supports zircon hosted biotite fractionation in the biotite granite (Clark et al. 1993).
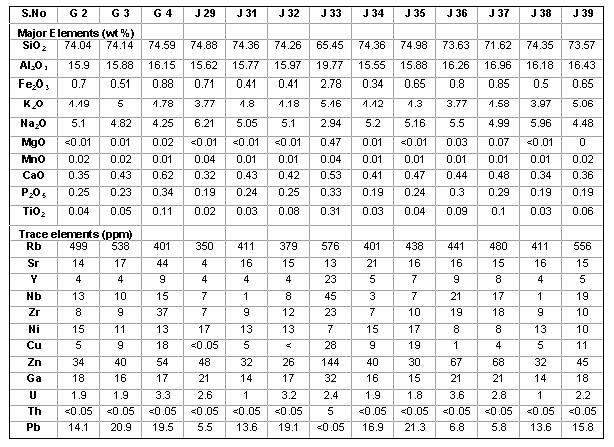
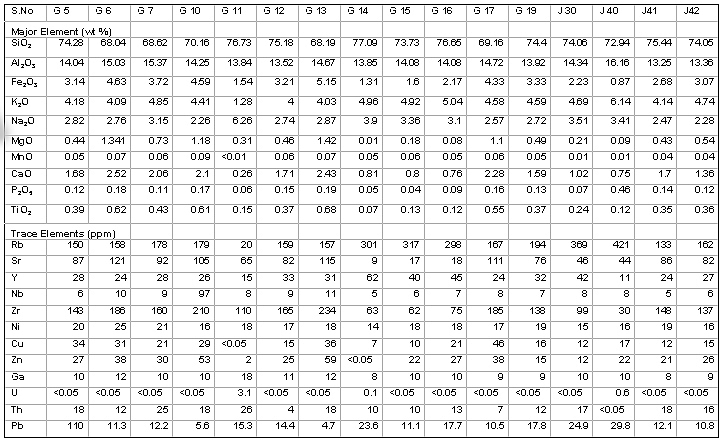
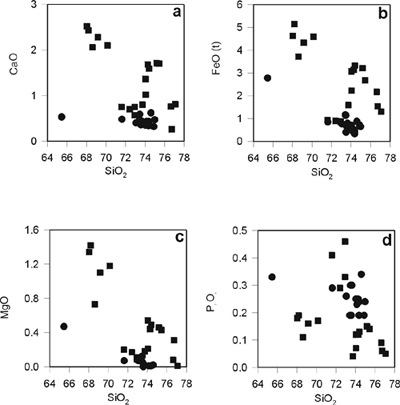
Steep depletion of Sr as a function of SiO2 in biotite granite indicate extensive fractionation of plagioclase (Fig.6 a), whereas Rb, which initially increases and then decreases (Fig.6 b), suggests K-feldspar grew at later stage. However, the overall pattern of Rb vs. Sr indicates that crystal fractionation is a viable mechanism for trace elemental variation in biotite granite (Fig. 6 c). Low Zr in leucogranite as compared to biotite granite indicates that there is little or no zircon (Fig. 6 d). It also follows that the degree of partial melting of a felsic source and low temperature resulted in an abundance of restitic zircon in the source. Spidergram plots of biotite granite and leucogranite show a similar trend, except for different abundances (Figs. 7 a & b). In biotite granite, Zr shows a positive trend indicating presence of zircon, whereas it has a negative anomaly in leucogranite. Also, P shows a large scattering in the biotite granite, but a restricted range and positive anomaly in leucogranite indicating precipitation of apatite within the accessory phase. The observed high negative anomaly of Th and variation in U probably indicates involvement of monazite as a residual phase in the generation of leucogranite.
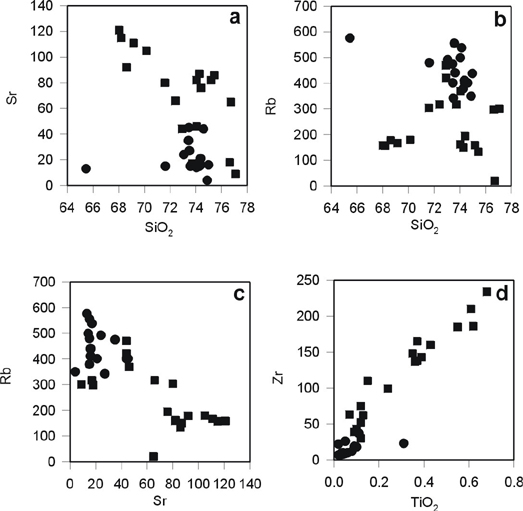
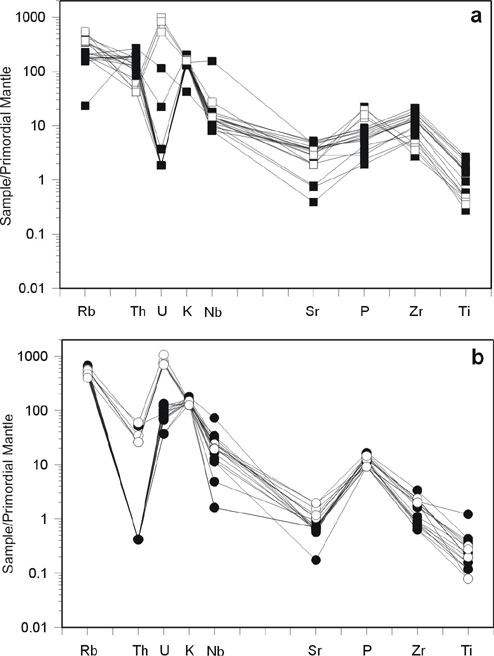
In this study, all the REE analyses (Table 3) were normalized to C1 Chondrite values and plotted in Figure 8. The Gangotri Granite shows lower LREE and HREE abundances as compared to the biotite granite. However, these show almost similar trends and are enriched in LREE and depleted in HREE. The BG show a steeper slope in LREE and a gentler slope in HREE giving a slightly concave trend revealing that these bodies are moderately fractionated in HREE with respect to LREE. However, in case of the LG, the HREE are more prominantly depleted. BG samples are also characterized by a strong negative Eu anomaly - an indication of fractionation of plagioclase. The Eu anomaly in case of LG is rather weak suggesting limited feldspar fractionation. Low Yb concentration and moderately fractionated REE pattern of LG suggest that these granites are derived from a crustal source, which is further supported by Rb vs. Y+Nb plot of Pearce et al. (1984), in which these bodies fall well within syncollisional domain (Fig. 9).
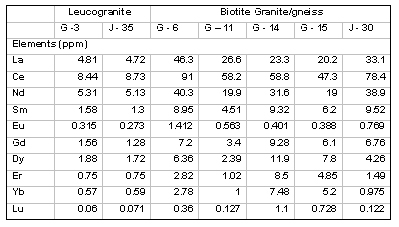
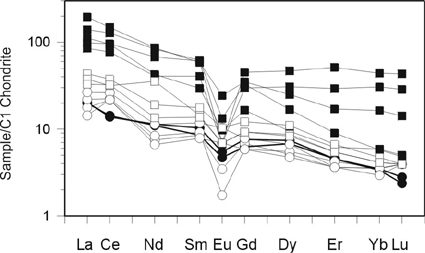
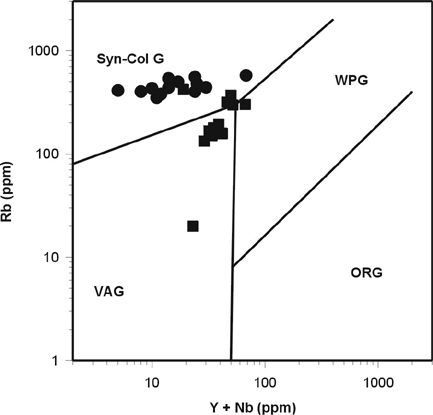
Whole-rock Rb/Sr isotopic ratios for biotite granite/gneiss and leucogranite have been attempted (Singh, 1986; Stern et al., 1989; Scaillet et al., 1990). The data given by these workers were recalculated and plotted on 87Rb/86Sr vs. 87Sr/86Sr plot by us. Two-error regression of data was made using a computer program, based on Wendt's Model II (Wendt, 1969).
87Rb/86Sr ratios of whole rock samples from the biotite granite/gneiss (Singh, 1986) have been plotted against 87Sr/86Sr values on Sr evolution diagram (Fig. 10 a). The two-error regression analysis of data yields an isochron age of 528.47±23.08 Ma with an initial 87Sr/86Sr ratio 0.710212±0.00033 and mean square of weighted deviation 0.78 and correspond to the Cambro-Ordovician (500±50 Ma) Pan-African magmatic event in the Himalaya. It may be of interest to note that Kwatra et al. (1999) have obtained 5-point whole rock Rb-Sr isochron age for the adjoining Raksham and Akpa granitoids of the Baspa-Sutlej Valleys as 453±8.84 and 477.29±29 Ma, respectively.
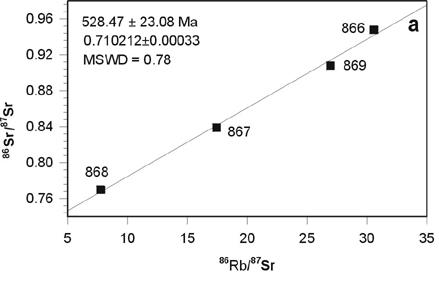
Stern et al. (1989) have published repeat Rb-Sr isotopic measurements on six whole-rock samples of the Gangotri Granite with an isochron age of 63.66±9.54 Ma with an initial 87Sr/86Sr ratio of 0.746645±0.00014 and MSWD value of 0.06 (Fig. 10 b). Later, Scaillet et al. (1990) obtained eight whole-rock Rb-Sr isotopic measurements from the Bhigupanth, Bhagirathi, Gangotri and Shivling plutons. The data obtained by them have a significant scattering on isochron diagram, but the regression line define an age of 51.25±9.09 Ma with MSWD value 1.92, and an initial 87Sr/86Sr ratio of 0.764525±0.00013 (Fig. 10 c).
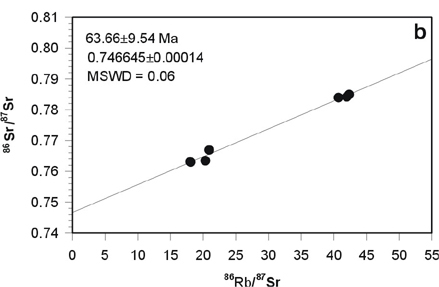
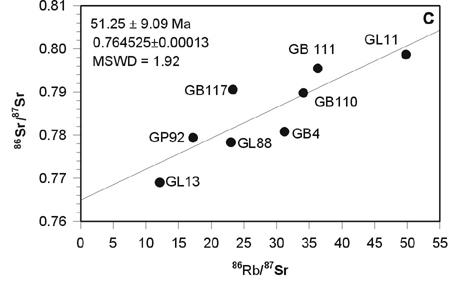
We have attempted to combine the data published by Stern et al. (1989) and Scaillet et al. (1990) in Figure 10 d. The data show a considerable scattering on the isochron diagram and the two o-error regression line yields an age of 57.05±6.72 Ma with an initial 87Sr/86Sr ratio 0.756109±0.0001 and a high MSWD value of 4.03 (Fig. 10 d). This shows a high level of isotopic variation, which could be either due to inhomogenity during initial stages of pluton formation or that the system became open after its emplacement and cannot be used to constrain the intrusive age of Gangotri Granite. Recently Harrison et al. (1997) obtained Th-Pb monazite ages for the Gangotri Granite from the proper Gangotri as 22.4±0.5 Ma and Shivling as 21.9±0.5 Ma. This matches the five-point Rb-Sr mineral isochron of 21.1±0.9 Ma by combining the whole rock with two feldspars, muscovite and tourmaline (Stern et al., 1989). Further, Searle et al. (1999) obtained a U-Pb age of 23.0±0.2 Ma for the Shivling leucogranite. The age of 22-23 Ma for the Gangotri Granite appears to be more appropriate and almost corresponds to the emplacement age of numerous Early Miocene Himalayan leucogranites.
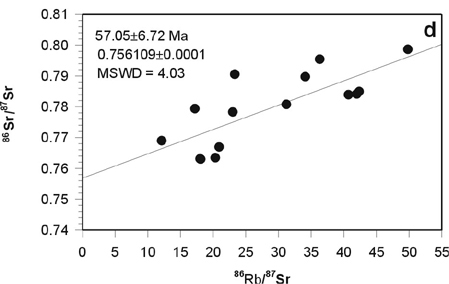
Systemic cooling and exhumation rates of the Gangotri Granite have been calculated recently after initial determination of muscovite K-Ar ages of 18.4±0.7 Ma and 18.9±1.3 Ma by Seitz et al. (1976) from a leucogranite sample from the Badrinath, and by Stern et al. (1989) from the Bhagirathi leucogranite, and a Rb-Sr mineral age of 21 Ma. Subsequent 40Ar/39Ar dates on muscovite separates from leucogranite at Gangotri yield plateau age of 17.9±0.1 Ma; and biotites from the biotite granite about 800 m above the sample gives a similar age of 18.0±0.1 Ma (Sorkhabi et al., 1999). A total of 15 apatite fission track ages from a cliff section above the Gangotri temple on the left bank of the Bhagirathi River between 2670 and 4370 m give an age range from 1.48±0.6 Ma to 2.41±0.52 Ma with a weighted mean of 2.0±0.52 Ma, exhumation and cooling rates of ~2 mm/yr and 63-70°C/Ma were calculated. These rapid cooling and exhumation rates contrast with 10-13°C/Ma cooling and 0.38 mm/yr exhumation rates from ~18 Ma to 2 Ma (Jain et al., 2000). Since the crystallization of the Gangotri Granite at 22 Ma, cooling and exhumation rates had been 80°C/Ma and ~ 3 mm/yr, respectively (Fig. 11).
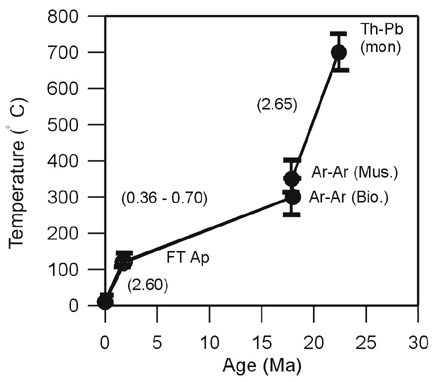
Petrogenesis of HHL
The peraluminous Himalayan S-type Cenozoic leucogranites within the HHC of the Zanskar-Garhwal-Nepal-Bhutan originated either partly or wholly in the lower crust, which is composed of metamorphics, migmatites and Early Paleozoic granites with distinct variations along the regional trend of the Himalayan orogen (cf. Le Fort et al., 1987; Daniel et al., 1987; Pognante et al., 1987; France-Lanard et al., 1988). These granitoids are generally more homogeneous large plutons intruding the higher stratigraphic and structural levels in the east. Different P-T-t paths have been observed in association with the leucogranite (Pognante, 1993), who argued the important role of liberated fluids during metamorphism; he also argued that decreasing pressure conditions from west to east across the Indian Plate is one of the main factors, controlling the increased abundance of leucogranites in Nepal. It has also been observed that there is a relatively high pressure anatexis in NW Himalaya, hence smaller leucogranite amounts are generated in contrast to Nepal. As such, a low degree of partial melting has partly inhibited the accumulation and ascent of the leucogranite melt, which therefore, remained in place to form in situ migmatites and leucogranite.
Different models have been put forward for the possible origin of the High Himalayan leucogranites. The tectonic model for the generation and emplacement of these granitoids has been suggested by Le Fort (1975, 1981, 1986) and Le Fort et al. (1987); this model combines thrusting on a continental scale along the Main Central Thrust (MCT) with development of inverted metamorphism and liberation of large quantities of fluids, thus causing anatectic melting of the crust. Thrusting along the MCT brings hot portions of the deep continental crust, the Tibetan slab, over the weakly metamorphosed Midland Formation (Lesser Himalayan rocks) in Nepal. This continuous process heats the Midland Formation from top to bottom and induces synkinematic inverted metamorphism. In turn, dehydration and decarbonation reactions release large amounts of fluids that rise above the MCT. In the portion of the Tibetan slab that is hot, these fluids induce anatexis and produce melts close to the minimum melt compositions. This melt is then emplaced at higher levels at the top of the Tibetan slab.
Searle and Fryer (1986) proposed that frictional heating was not compatible due to the high water and volatile contents and requires dry melt. They suggested that crustal anatexis caused migmatization and subsequent intracontinental subduction liberated large quantities of fluids, which induced melting. These boron-rich fluids induced the melting by lowering the solidus, and therefore, making the magma less viscous. This melt crystallized tourmaline and muscovite with garnet, feldspar, and quartz.
The mechanism of intrusion for the Higher Himalayan leucogranite is influenced by such factors as composition, volume, viscosity, water content of the magma and the state of stress in the Higher Himalaya. It has been argued that the emplacement mechanism of the leucogranites is mainly related to their level in crust (Searle et al., 1988; Searle and Rex, 1989; Searle, 1991). According to Searle (1991), deeper structural levels are associated with partial melting that produces migmatites and layered granite sheets as well as remobilization of granite, whereas mid-crustal levels are characterized by sheet-like intrusions along fluid-rich ductile shear zones as a result of melt-enhanced deformation (Hollister and Crawford, 1986). High structural levels are characterized by migrated plutons that originate from deep levels in the crust and have intruded upwards, cross cutting the regional metamorphic isograds.
The simplest model for the leucogranite formation results from anatexis of continental crust during Himalayan collision tectonics, which resulted in crustal thickening and isostatic readjustments. It has resulted in a depression at the base of the continental block, which is forced into a higher temperature environment, causing melting of the lower crustal rocks.
Discussions
The Higher Himalayan Leucogranite (HHL) Belt is a result of the collision-related magmatism with a strong peraluminous character with muscovite and tourmaline along with uniform minimum-melt compositions (Kai, 1981; Le Fort et al., 1987; Inger and Harris, 1993; Harris et al., 1993; Gulliot and Le Fort, 1995, Searle et al., 1997). Two-mica leucogranites are found in most orogenic belt as a result of syntectonic anatexis processes in the crust. The regional Barrovian Himalayan metamorphism gave an anti-clockwise P-T path (for summary see Gulliot et al., 1999 and Jain et al., 2002) deduced from medium- and high-grade zones due to crustal thickening. Thickening of the crust induced a tectonic denudation by collapse of the upper terrain and short-lived tectonic burial followed by exhumation. The exhumation during Miocene exhumation caused the decompression melting and anatexis.
This metamorphism led to the formation of anatectic migmatites in the upper level of the HHC and the intrusion of leucogranite magma as both insitu incipient melting and emplacement of large-scale sheets and sills of crustally derived intrusive. The observed isotopic signatures (Sr, Nd and Pb) on the HHL belt (Vidal, 1978; Denial et al., 1987; Gareipy et al., 1985) along with experimental data (Scaillet et al., 1995; Patinio Douce and Harris, 1998) indicate the source could be metasedimentary. Geochronological data on the HHL belt suggest that they have been emplaced between a older 24 Ma and a younger 17 Ma episode (Noble and Serale, 1995; Harrisson et al., 1997, 1999; Searle et al, 1997, 1999). Though the ages vary, the major phase of leucogranite occurred by dehydration melting between 24-22 Ma.
The formation of the HHL belt is a matter of debate among the workers. Mainly three interpretations have been put forward: (1) the HHL formed due to the fluid-induced anatexis of MCT hanging-wall rocks, triggered by devolatilization of overthrust footwall rocks in concert with shear heating (Le fort, 1975; Fance-Lanord and Le Fort, 1988; England et al., 1992). Le Fort (1975) proposed that fluid introduced into the overlying HHC from the dehydrating footwall led to the formation of the HHL melt via minimum melting reactions (also Inger and Harris, 1992). (2) the HHL formed by dehydration melting caused by decompression during slip on the South Tibetan Detachment System (STDS) (Harris et al., 1993; Inger and Harris, 1993; Harris and Massey, 1994; Gulliot and Le Fort, 1995; Searle et al., 1997). This indicates that the anatexis immediately followed substantial displacement along the STDS but may not have been synchronous with motion on the MCT. Harris et al. (1993) proposed that the HHL were produced by high-temperature muscovite dehydration melting and fluids became mobile only after tectonic decompression (via the STDS). Decompression melting along the footwall of STDS has been cited as the main process responsible for the formation of the leucogranite. Furthermore, recent experimental evidence indicates that water-saturated melting of the Himalayan source rock produced trondhjemite melt rather than leucogranite (Patino Douce and Harris, 1998). (3) the HHL belt could be linked to continous shear heating and cuts through Indian Supra-crustal rocks that were basement during the initial stages of the Indo-Asia collision (Harrisson et al., 1997, 1999). Decompression above MCT cannot trigger melting rather this mechanism could work in concert with fractional heating along a thrust surface; anatexis resulted from vapour-absent muscovite dehydration melting of the HHC. The HHC represent the melting properties of the source region by averaging the experimentally determined melting relationship for various metasedimentary composition (Gardien et al., 1995), which is closely related to muscovite and biotite dehydration melting (Le Preton and Thompson, 1988).
Summary & Conclusions
The depleted Sr and CaO, high silica and Na2O content together with the narrow range of major and trace elemental abundance in LG implies that there is not much variation in the degree of partial melting. A tight felsic compositional range may represent minimum melt composition of a felsic source with abundant feldspar, thus precludes the possibility of the melt generation from pelitic meta-sedimentary source. The high-grade metasedimentary rocks and associated migmatites near the LG also show contrasting geochemistry. The observed linearity in binary variation diagrams and sub-parallel nature of the REE and spider-diagram pattern of the LG with the BG suggests that the BG is the most likely source for LG melt. This is also consistent with field observation of the two granitoids bodies in this area.
The albitic plagioclase in LG suggests incongruent low fraction partial melting of BG, in which the Na-rich component of plagioclase partitioned into melt leaving behind Ca-rich more anorthitic plagioclase in the residue. This has also resulted in depleted Sr content in LG, which is preferentially retained by restitic plagioclase. Similarly, the incongruent melting of K-feldspar, would produce K-rich melt and would also be enriched in Rb as observed in LG. In the case of a higher degree of partial melting, these signatures would get diluted and therefore, we suggest that the LG was produced by lower degree of partial melting probably at shallower depth and low temperature. The pH2O requirement for low temperature melting was probably met internally by dehydration reactions of abundant hydrous phases like biotite and muscovites and intergranular water dissolved at grain boundaries (Pichavant et al. 1988, Pichavant and Montel, 1988). The observed low Zr further substantiates this and hence more depleted HREE that results from the non-involvement of refractory minerals like zircon, and probably also monazite, in the melting process.
The problem of separation of low fraction felsic melts from the source due to high viscosity has been discussed in detail by Wickman, (1987). It is suggested that a interconnected low fraction felsic melt can only be separated from the source by compaction (Jurewicz and Watson, 1984, 1985). Since the LG are related to syn-collisional events, the compactional or shearing stress enabled the felsic melt fraction to separate from the source region.
Acknowledgements
The present work was carried out under the Department of Science and Technology sponsored projects by SS and AKJ over the years. It took us a long time to finalize this manuscript through the opportunity given by Virtual Explorer in the form of an edited volume on Granitoids of Himalayan Collisional Belt. We thank the Director of the Wadia Institute of Himalayan Geology for use of XRF and ICP facilities for geochemical analysis. We also thank Drs. K.R. Gupta, RM. Manickavasagam, M.S. Rathi, R.S. Sharma, Rasoul Sorkhabi, Nand Lal for constant support and encouragement throughout the work. The review of this article by Mary Leech and Gary Solar helped in improving the manuscript.
References
- Auden, J.B. (1935) Traverses in the Himalaya. Rec. Geol. Surv. India, v. 69, pp. 123-167.
- Auden, J.B. (1949) General report of G.S.I. for 1939. Rec. Geol. Surv. India, v. 78, pp. 74-78.
- Chappel, B.W. and White, A. J. R. (1992) I- and S- type granites in Lachland Fold belt. Trans. Royal. Soc. Edinberg (ES) v. 83, pp. 1-26.
- Clark, DB., Macdonald, MA., Reynolds, PH., and Longstaffe, FJ., (1993). Leucogranites in the Lachlan fold belt. Trans. R. Soc. Edinburg (ES), v. 83, pp. 1-26.
- Debon, F., Le Fort, P., Sheppard, S.M.F. and Sonet, J. (1986) The four plutonic belts of the Transhimalaya-Himalaya: A chemical, mineralogical, isotopic and chronological synthesis along a Tibet-Nepal section. J. Petrol., v. 27, pp. 219-250.
- Deniel, C., Vidal, P., Fernandez, A., Le Fort, P. and Peucat, J.J. (1987) Isotopic study of the Manaslu granite (Himalaya, Nepal): Inferences on the age and source of Himalayan leucogranites. Contrib. Miner. Petrol., v. 96, pp. 78-92.
- England, P., Le Fort, P., Molnar, P. and Pecher, A. (1992) Heat source for Tertiary metamorphism and anatexis in the Annapurna-Manaslu region (central Nepal). J. Geophys. Res., v. 97, pp. 2107-2128.
- France-Lanord , C. and Le Fort, P. (1988) Crustal Melting granite and genesis during the Himalayan collision orogenesis. Trans. R. Soc. Edinburgh (Earth Sci.), v. 79, pp. 183-195.
- France-Lanord, C., Sheppard, S.M.F. and Le Fort, P. (1988) Hydrogen and oxygen isotope variations in the High Himalaya peraluminous Manaslu leucogranite: evidence for heterogeneous sedimentary source. Geochim. Cosmochim. Acta, v. 52, pp. 513-526.
- Gardien, V., Thompson, A.B., Grujic, D. and Ulmer, P. (1995) Experimental melting of biotite + plagioclase + quartz ± muscovite assemblages and implications for crustal melting. Jour. Geophys. Res., v. 100, pp. 15581-15591.
- Gareipy, C., Allegre, C.J. and Xu, R.H. (1985) The Pb-isotope geochemistry of granitoids from the Himalaya-Tibet collision zone: implications for crustal evolution. Earth Planet. Sci. Lett., v. 74, pp. 220-234.
- Griesbach, C.L., 1893. Notes on the Central Himalaya. Rec. Geol. Surv. India, v. 26, pp. 19-25.
- Gulliot, S. and Le Fort, P. (1995) Geochemical constraints on the bimodal origin of High Himalayan leucogranites. Lithos, v. 35, pp. 231-234.
- Gulliot, S., Cosca, M., Allemand, P. and Le Fort, P. (1999) Contrasting metamorphic and geochronologic evolution along the Himalayan belt. In: Macfarlane, A., Sorkhabi, R.B. and Quade, J. (Eds.) Himalaya and Tibet: Mountain roots to mountain tops. Geol. Soc. Amer. Spec. Paper 328, pp. 117-128.
- Harris, N. and Massey, J. (1994) Decompression and anatexis of Himalayan metapelites. Tectonics, v. 13, pp. 1537-1546.
- Harris, N., Inger, S. and Massey, J. (1993) The role of fluids in the formation of High Himalayan leucogranites. In: Treloar, P.J. and Searle, M.P. (Eds.) Himalayan Tectonics. Geol. Soc. London Spec. Publ. No. 74, pp. 391-400.
- Harrison, T. M., Lovera, O.M. and Groove, M. (1997) New insights into the origin of two contrasting Himalayan granite belts. Geology, v. 25, pp. 899-902.
- Harrison, T.M., Grove, M., Lovera, O.M., Catlos, E.J. and D’Andrea, J. (1999) The origin of Himalayan anatexis and inverted metamorphism: Models and constraints. J. Asian Earth Sci., v. 17, pp. 755-772.
- Heim, A. and Gansser, A (1939) Central Himalaya- Geological observations of Swiss Expedition 1936. Mem. Soc. Helve. Sci. Nat., v. 73, 245 pp.
- Hodges, K.V. (2000) Tectonics of the Himalaya and southern Tibet from two decades perspectives. Geol. Soc. Amer. Bull., v. 112, pp. 324-350.
- Hodges, K.V. , Parrish, R.R., Housh, T.B., Lux, D.R., Burchfiel, B.C., Royden, L.H. and Chen, Z. (1992) Simultaneous Miocene extension and shortening in the Himalayan orogeny. Science, v. 258, pp. 1466-1470.
- Hollister, L.S. and Crawford, M.L. (1986) Melt enhanced deformation: a major tectonic process. Geology, v. 14(7), pp. 558-561.
- Inger, S. and Harris, N.B.W. (1992) Tectonothermal evolution of the High Himalayan crystalline sequence, Langtang Valley, northern Nepal. J. Metam. Geol., v. 10, pp. 439-452.
- Inger, S. and Harris, N. (1993) Geochemical constraints on leucogranite magmatism in the Langtang valley, Nepal Himalaya. J. Petrol., v. 34, pp. 345-368.
- Jain, A. K., Kumar, D., Singh, S. Kumar, A. and Lal, N. (2000) Timing, quantification and tectonic modeling of Pliocene Quaternary movements in the NW Himalaya: evidences from fission track dating. Earth Planet. Sci. Lett., v. 179, pp. 437-451.
- Jain, A. K., Singh, S. and Manickavasagam, RM. (in press) Himalayan Collision Tectonics, Bhagirathi Valley Garhwal. Gondwan Research Group Memoir No. 2002
- Johannes, W. and Holtz, F. (1990) Formation and composition of H2O-undersaturated granitic melt. In: Asworth, J.R. and Brown, M. (eds) High temperature metamorphism and crustal anatexis, London; Unwin Hyman, pp. 87-104.
- Jurewicz, W. and Watson, EB. (1984). Distribution of partial melt in felsic system : Importance of surface energy. Contrib. Min. Petrol., v. 85, pp. 125-129.
- Jurewicz, W. and Watson, EB. (1985). The distribution of partial melt in granitic system : the application of liquid phase sintering theory. Geochim. Cosmochim. Acta, v. 49, pp. 1109-1121.
- Kai, K. (1981) Rb-sr geochronology of the rocks of the Himalayas, Eastern Nepal. Part I. The metamorphic age of Himalayan Gneiss. Memoirs of the Faculty of Science, Kyoto University, Series of Geology and Mineralogy, v. 47, pp. 135-148.
- Kwatra, S. K. Singh, S., Singh, V. P., Sharma, R. K., Rai, B. and Kishor, N. (1999) Geochemical and geochronological characteristics of the Early Palaeozoic Granitoids From Sutlej-Baspa Valley, Himachal Himalaya. In: Jain, A.K. and
- Manickavasagam, RM. (Eds.) Geodynamics of the NW-Himalaya, Gondwana Res. Group Mem. 6, pp. 145-158.
- Le Fort, P. (1975) Himalayas: The collided range, Present knowledge of the continental arc. Amer. J. Sci., v. 275, pp.1-44.
- Le Fort, P. (1981) Manaslu leucogranite: A collision signature of the Himalaya. A model for its genesis and emplacement. J. Geophys. Res., v. 86, pp. 10,545-10,568.
- Le Fort, P. (1988) Granites in the tectonic evolution of Himalaya, Karakoram and southern Tibet. In: Tectonic evolution of the Himalayas and Tibet. Phil. Trans. R. Soc. London, v. 326, pp. 281-299.
- Le Fort, P., Cuney, M., Deniel, C., Lanords, C.F., Sheppard, N.F., Upreti, B. N. and Vidal , P. (1987) Crustal generation of the Himalayan leucogranite. Tectonophysics, v. 134, pp. 39-57.
- Le Preton, N. and Thompson, A.B. (1988) Fluid-absent (dehydration) melting of biotite in metapelites in the early stages of crustal anatexis. Contrib. Mineral. Petrol., v. 99, pp. 226-237.
- McMahon, C.A. (1884) Microscopic structures of some Himalayan granites and gneissose granites. Rec. Geol. Surv. India, v. 17, pp. 53-73.
- Noble, S.R., and Searle, M.P. (1995) Age of crustal melting and leucogranite formation from U-Pb zircon and monazite dating in the Western Himalaya, Zanskar, India. Geology, v. 23 (12), pp. 1135-1138.
- Odell, N.E. (1983) On the occurrence of granites in the Himalayan Mountains. In: Shams, F.A. (Ed.) Granites of Himalaya, Karakoram and Hindukush. pp. 1-10.
- Pande, K. (1999) Present status of K-Ar and 40Ar-39Ar data from the Himalaya In: Jain, A.K. and Manickavasagam, R.M. (Eds.) Geodynamics of the NW-Himalaya. Gondwana Res. Group Mem. No. 6, pp. 237-244.
- Pant, R. (1986) Petrochemistry and petrogenesis of the Gangotri granite and associated granitoids, Garhwal Himalaya. Unpubl. Ph. D. Thesis, Univ. Roorkee, India. 120 pp.
- Pant, R. and Dave, V.K.S. (1992) Petrochemistry and petrogenesis of tourmaline-muscovite leucogranite of Gangotri, Uttarkashi district, Garhwal. Bull. Indian Geol. Assoc., v. 25(1-2), pp. 159-167.
- Patino Douce, A.E. and Harris, N. (1998) Experimental constraints on Himalayan anatexis. J. Petrol., v. 39, pp. 904-911.
- Pearce, J.A., Harris, N.B.W. and Tindle, A.G. (1984) Trace element discrimination diagram for the tectonic interpretation of granitic rocks. J. Petrol., v. 25, pp. 956-983.
- Pognante, U. (1993) Different P-T-t paths and leucogranite occurrences along the High Himalayan Crystallines: Implications for subduction and collision along the northern Indian margin. Geodynamica Acta, v. 6(1), pp. 5-17.
- Pognante, U., Genovese, G., Lombardo, B. and Rosetti, P. (1987) Preliminary data on the High Himalayan Crystallines along the Padam-Darcha traverse south eastern Zanskar, India. Rend. Soc. Ital. Miner. Petrol., v. 42, pp. 95-102.
- Pichavant, M. and Montel, JM. (1988). Petrogenesis of a two mica ignimbrite suite : the Macusani volcanics, SE Peru. Trans. R. Soc. Edinburg (ES), v. 79, pp. 197-208.
- Pichavant, M., Kontak, JD., Briqueu, L., Valencia herrara, J. and Clark, AH. (1988). The Macusani volcanics, SE Peru, II. Geochemistry and origin of a felsic peraluminous magma. Cont. Min. Petrol., v. 100, pp. 325-338.
- Rathi, MS., Khanna, PP., Mukherjee, PK., and Saini, NK, (1996) Evaluation of REE determination in geological samples by inductively coupled plasma spectroscopy, Jour. Geol. Soc. India, v. 48, pp. 325-330.
- Saini et al (2002)
- Scaillet B., France-Lanord C. and Le Fort P. (1990) Badrinath-Gangotri plutons (Garhwal Himalaya): Petrological and geochemistry evidence for fractionation processes in a high Himalayan leucogranite. J. Volcan. Geotherm. Res., v. 44 (1/2), pp. 163-188.
- Scaillet B., Pichavant, M. and Roux, J. (1995) Experimental crystallization of leucogranite magma. Jour. Petrol., v. 36, pp. 663-705.
- Searle, M.P. (1991): Geology and Tectonics of the Karakoram Mountains. John Wiley & Sons, Chichester, 358 pp.
- Searle, M.P and Fryer, B.J. (1986) Garnet, tourmaline, and muscovie-bearing leucogranites, gneisses and migmatites of the Higher Himalaya from Zanskar, Kulu, Lahoul and Kashmir In: Cowards, M.P. and Ries, A.C. (Eds.) Collision Tectonics. Geol. Soc. London Spec. Publ. No. 19, pp. 185-201.
- Searle, M.P. and Rex, A.J. (1989) Thermal model of the Zanskar Himalaya. J. Metam. Geol., v. 7, pp. 124-134.
- Searle, M.P., Cooper, D.J.W and Rex, A.J. (1988) Collision tectonics of the Ladakh-Zanskar Himalaya. Phil. Trans. R. Soc. London, v. A326, pp. 117-150.
- Searle, M.P. Metcalfe, R.P., Rex, A.J. and Norry, M.J. (1993) Field relations, petrogenesis and emplacement of the Bhagirathi leucogranite, Garhwal Himalaya In: Treloar, P.J. and Searle, M.P. (Eds.) Himalayan Tectonics. Geol. Soc. London Spec. Publ. No. 74, pp. 429-444.
- Searle, M.P., Parrish, R.R., Hodges, K.V., Hurford, A., Ayres, M.W. and Whitehouse, M.J. (1997) Shishma Pangma Leucogranite, South Tibetan Himalaya: field relations, geochemistry, age, origin and emplacement. J. Geol., v. 105, pp. 295-317.
- Searle, M.P., Noble, S.R., Hurford, A.J. and Rex, D.C. (1999) Age of crustal melting, emplacement and exhumation history of the Shivling leucogranite, Garhwal Himalaya. Geol. Mag, v. 136 (5), pp. 513-525.
- Seitz J.R. Tiwari, A.P. and Obradovich, K. (1976) A note on the absolute age of the tourmaline-granite, Arwa Valley Garhwal Himalaya-Geol. Surv. India Misc. Publ. v. 24 pp. 332-337.
- Singh, R. P., 1986. Rb-Sr dating and Sr isotopic studies of some granites and gneisses of Kumaun and Garhwal Himalaya, U.P., India. Unpublished Ph.D. Thesis, Panjab University, Chandigarh.
- Singh, S. (2001) Status of geochronological studies in Himalaya: a review. J. Indian Geophys. Union., v. 5 (1), pp. 57-72.
- Sorkhabi, R.B., Stump, E., Foland, K.A. and Jain, A.K. (1996) Fission-track and 40Ar/39Ar evidence for episodic denudation of the Gangotri granites in the Garhwal Higher Himalaya, India. Tectonophysics, v. 260, pp. 187-199.
- Sorkhabi, R.B., Stump, E., Foland, K.A. and Jain, A.K. (1999) Tectonic and cooling history of the Garhwal Higher Himalaya (Bhagirathi valley): constraining from thermochronological data In: Jain, A.K. and Manickavasagam, R.M. (Eds.) Geodynamics of the NW-Himalaya. Gondwana Res. Group Mem. No. 6, pp. 217-235.
- Stern, C. R., Kligfield, R., Schelling, D., Virdi, N.S., Futa, K., Peterman, Z. E. and Amini, H. (1989) The Bhagirathi leucogranite of the High Himalaya (Garhwal, India): Age, petrogenesis and tectonic implications. Geol. Soc. Amer. Specl. Paper 232, pp. 33-45.
- Vidal, Ph. (1978) Rb-Sr systematics in granites from Central Nepal (Manaslu): significance of the Oligocene age and high 87Sr/86Sr ratio in Himalayan Orogeny: comment. Geology, v. 6(4), pp. 196-200.
- Wadia, D.N. (1928) The geology of the Poonch State, Kashmir and adjacent parts of the Panjab. Mem. Geol. Surv. India, v. 51, 233 pp.
- Wadia, D.N. (1957) Geology of the India. McMillan & Co., London, 536pp.
- Wendt, I. (1969) Derivation of the formula for a regression line based on a least square analysis. Internal. Rep. Bundesantalt Fur Bodenforschung, Hanover, West Germany.
- Wickham, SM. (1987) The segregation and emplacement of granitic magma., Jour. Geol. Soc. London, v. 144, pp. 281-297.
- Zen, E-AN (1986), aluminium enrichment in silicate melts by fractional crystallization : some mineralogic and petrographic constraints, Jour. Petrol., v. 27, pp. 1095-1117.